Tools and Techniques of Molecular Biology
Recombinant DNA Technology
Learning Objectives
When you have mastered the information in this chapter, you should be able to:
- Describe the characteristics of plasmids/ cloning vectors
- List in words or indicate in a drawing the important features of a plasmid vector that are required to clone a gene. Explain the purpose of each feature.
- Explain how vectors are used in cloning a gene.
- Explain what are expression vectors and how they are used.
- Explain how to use restriction enzymes to create a recombinant plasmid.
- What properties of the DNA restriction fragments enable ligation of these fragments?
- Explain what role do restriction enzymes have in nature?
- Explain how DNA ligase is used to create a recombinant plasmid
- Describe possible recombinant plasmids that form when ligating a restriction digest
- Design a cloning experiment/strategy when given vector sequences and insert sequences
- Predict or troubleshoot the reasons for a ‘failed’ cloning experiment.
- Due to a mishap in the lab, bacteria carrying a plasmid with a kanamycin-resistant gene and bacteria carrying a plasmid with an ampicillin-resistance gene were accidentally mixed together. How would you design an experiment allowing you to sort out the two kinds of bacteria?
- Explain the use of reporter gene assays.
- Outline an experiment using reporter gene assays to identify regulatory elements in promoter sequences of genes (prokaryotic and eukaryotic)
Introduction
It is likely you know somebody who has diabetes (a disease that occurs when a person’s blood glucose [sugar] is too high).
In certain types of diabetes, the body is unable to manufacture or produce insulin, the protein hormone needed to manage blood glucose levels.
In Chapter 1 and Chapter 2, you learned about the breakthrough research that led to the isolation of insulin, first from dogs and subsequently from pancreases of cows, and subsequent demonstration that purified insulin when injected in humans was able to treat the symptoms.
By 1923, insulin had become widely available in mass production, and Banting and Macleod were awarded the Nobel Prize in medicine. Diabetes was no longer a life-shortening disease. While these methods were effective, using animal-produced proteins sometimes resulted in adverse reactions, and making large enough quantities was difficult. This all changed with the advent of recombinant DNA technology.
DNA sequences encoding human insulin could be introduced into bacteria, which then make the hormone along with all of its bacterial proteins. In 1982, human insulin was the first commercially successful product made by recombinant DNA technology.
Molecular Cloning
Recombinant DNA molecules are DNA molecules made from joining together of DNA fragments from different sources. The applications of recombinant DNA molecules are many! Recombinant DNA molecules are used to remove (knock out) or add (knock-in) specific genes, to study gene expression.
This use of recombinant DNA technology is termed DNA cloning or Molecular Cloning-where multiple copies of the DNA are made via replication by the host cell DNA synthesis processes. The inserted genes can also be transcribed and translated to form protein as we saw for therapeutic purposes or to generate chimeric proteins to study novel functions of proteins or protein domains.
What do we need to generate recombinant DNA molecules?
- We need a way to isolate the gene/ DNA sequence (for example, a gene for a human therapeutic protein) away from the rest of the genome. This would involve cutting DNA in a reproducible manner and procedures for viewing the DNA fragments.
- We need a way to ‘paste’ this DNA into a vehicle (vector) to generate the recombinant molecule.
- The host cell should be able to replicate (make copies) of the recombinant molecule using host cell replication machinery.
- We need procedures for introducing the recombinant DNA molecule into the appropriate cell (eukaryotic or prokaryotic)
Three important tools that made the development of recombinant DNA molecules possible came from studying the basic science of prokaryotic biology.
First was the identification of a class of DNA-cutting enzymes called “restriction endonucleases” in bacterial cells. Second, was the identification of extrachromosomal DNA found in bacteria called ‘Plasmids’. Third was the knowledge that bacteria can take up DNA from exogenous sources or the medium as demonstrated by Griffith Transformation experiments and Avery and MacLeod experiments!
We will first discuss the identification and restriction of endonucleases.
Digestion of DNA with restriction endonucleases, pasting with DNA ligases
Restriction endonucleases (restriction enzymes) are enzymes produced by bacteria as a protection mechanism to cut and destroy foreign cytoplasmic DNA that is most commonly a result of bacteriophage infection.
Stewart Linn and Werner Arber discovered restriction enzymes in their 1960s studies of how E. coli limits bacteriophage replication or infection. (Read the Link to learning).
Restriction enzymes serve as molecular scissors recognizing a specific sequence in the DNA called restriction sites. The sites are usually palindromic, typically between four to six base pairs in length.
Palindromic sequences are the same sequence forwards and backward. Some examples of palindromes: RACE CAR, CIVIC.
For DNA, 2 strands run antiparallel to each other. Therefore, the reverse complement of one strand is identical to the other. This means that the sequence of the recognition site when read in the 5‘ to 3‘ direction for the top strand is the same as that of the bottom strand.
Upon binding this sequence, the enzyme cuts both strands of the DNA generating a double-stranded break.
However, based on where the enzyme cuts different kinds of ends are generated. Some enzymes cut asymmetrically leaving an overhang at the 5’ end of one strand of the duplex; some leave an overhang at the 3’ end. These ends are referred to as “sticky ends”. (Figure 2B)
Some enzymes cut symmetrically leaving no overhanging sequence overhangs and generating “blunt ends”(Figure 2A)
This distinction in cutting is important because “sticky ends” are self-complementary (hence the name sticky!)
Molecules with complementary or compatible sticky ends can easily anneal (or stick to one another) forming hydrogen bonds between complementary bases, at their overhangs.
With the help of an enzyme we are already familiar with, DNA Ligase the 2 pieces of DNA are joined together through covalent bonding, making the molecule a continuous double strand.
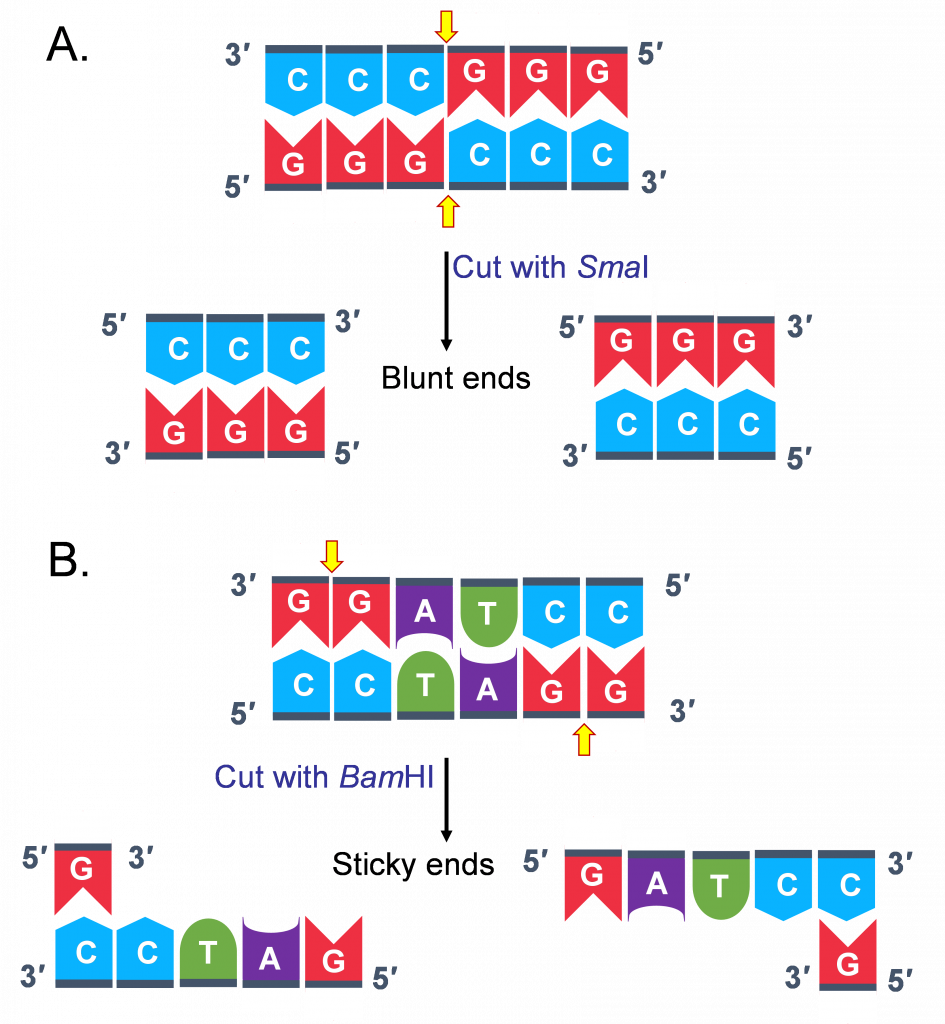
With a combination of RE and DNA ligase, any two fragments regardless of their origin (animal, plant, fungal, bacterial) can be joined in vitro to form recombinant molecules, especially if they have compatible sticky ends.
COMPLETE Lecture Activities Below
TRY THIS ACTIVITY:
Link to Learning: Discovery of Restriction Endonucleases.
Why would bacteria have enzymes that could cut up DNA?
The discovery of this special class of enzymes came from early work in the 1950s on certain strains of bacteria. Scientists observed that certain strains of E. coli, a common bacterium found in the human gut, were resistant to infection by bacteriophage—a virus that infects bacteria by injecting its DNA into the cell and commandeering the host cell’s molecular processes to make more bacteriophage. This peculiar (at the time) phenomenon was called ‘Host-Restriction”. 1960s, Werner Arber was investigating the possible mechanisms and observed a dramatic change in the bacteriophage DNA after it invaded these resistant strains of bacteria: the bacteriophage DNA was degraded and cut into pieces! To explain the resistance of certain bacterial strains to bacteriophage infection, Arber hypothesized that bacteriophage-resistant bacterial cells might express a specific enzyme that degrades only invading bacteriophage DNA, but not their own DNA. Further investigation of this primitive bacterial “immune system” led to the discovery of restriction enzymes, proteins that recognize cut DNA at specific sequences called restriction sites. Thus, they restrict the growth of bacteriophages by recognizing and destroying the phage DNA. Read the following article to learn how bacteria protect their DNA from being chewed up.
(https://www.nature.com/scitable/spotlight/restriction-enzymes-18458113/)
Plasmids or Vectors
Bacteria carry a single circular genome (chromosome) that carries all the information needed by the organism to survive and reproduce. In the 1950s it was discovered that many bacteria carry additional small DNA molecules, ranging in size from 1,000 to 200,000 base pairs that could replicate independently from the chromosomal DNA. These plasmids provided a selective advantage to the bacteria by carrying genes that encoded proteins that provided resistance to antibiotics. These extrachromosomal DNA molecules were called Plasmids.
Plasmids thus carried two features that were recognized as useful to enable molecular cloning:
- They have an origin of replication, which allows the plasmid to produce many copies (replicate) inside of a bacterial cell. Additionally, as mentioned above they replicate at a higher frequency than the bacterial chromosome, which means there could be thousands of copies of plasmid within a single cell.
- They carry a gene for antibiotic resistance, that provides a mechanism to select for a small percentage of bacterial cells that have taken up the plasmid
Scientists took advantage of these plasmids and repurposed them to use as tools to clone, transfer, and manipulate genes. Plasmids that are used experimentally have been repurposed to make them suitable for cloning and called vectors.
****** Terminology alert: Often the terms plasmid and vector are used interchangeably************
Vectors (plasmids) used by scientists today come in many sizes and vary broadly in their functionality. The greatest variety of cloning vectors has been developed for use in the bacterial host E. coli.
They all however must have a minimum of three features
1) An origin of replication
2) A selectable marker (antibiotic resistance gene)
Cells that take up the plasmid will be able to grow in the presence of the antibiotic. If bacterial cells to which the plasmid has been added are plated on agar containing the antibiotic, the cells that took up the plasmid will be able to grow, while the others will not. Usually, the percentage of bacterial (or other host) cells that take in externally added DNA is low. Selection allows for easy identification of those that do contain the plasmid.
3) A cluster of restriction enzyme sites called a Multiple cloning site (MCS).
The MCS enables researchers to introduce any piece of DNA into the vector by restriction enzymes digestion and ligation. As a general rule, the restriction sites in the MCS are unique and not located elsewhere in the plasmid backbone, which is why they can be used for cloning by restriction enzyme digestion but when designing a cloning experiment a detailed ‘Restriction Map’ can be obtained”.
Watch this video embedded below or go to this YouTube link from Addgene
Building a recombinant DNA molecule: The workflow
How do scientists use the two tools above to generate a recombinant DNA molecule?
Step 1. Isolate DNA of interest (called insert in a cloning experiment)
Step 2. Identify cloning vector/plasmid
Step 3.
– once the target and vector DNA have been identified, both types of DNA are cut using restriction endonucleases.Step 4. Purify the cut fragments: U
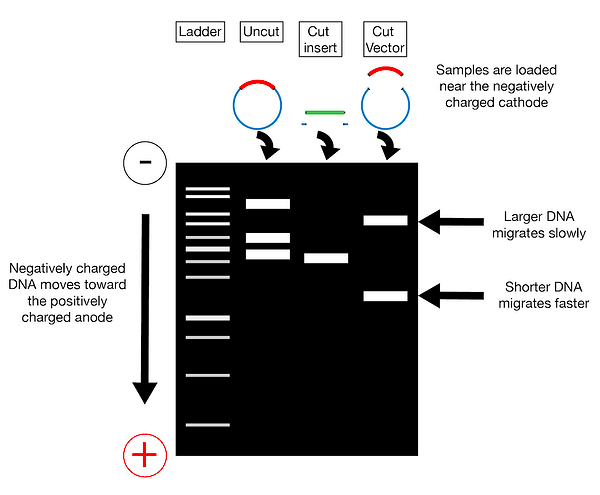
Step 5. Combine purified target and vector DNA – the two types of DNA are combined in a single tube with the addition of DNA ligase and appropriate buffer. This results in the creation of recombinant DNA.
Step 6. Introduce recombined molecule into the host cell
Once the target DNA has been stably combined with vector DNA, the recombinant DNA must be introduced into a host cell, in order for the genes to be replicated or expressed. There are different methods for introducing the recombinant DNA, largely depending upon the complexity of the host organism.
In the case of bacteria, transformation is often the easiest method, using competent cells to pick up the recombinant DNA molecules. Alternatively, electroporation can be used, where the cells are exposed to a brief pulse of high–voltage electricity causing the plasma membrane to become temporarily permeable to DNA passage.
Step 7. Grow the bacteria and plate on agar plates that contain antibiotics. Bacterial cells carrying the plasmid will grow, multiply, and form visible colonies because the plasmid carries the gene that provides resistance to the antibiotic. If your vector had an Ampicillin Resistance Gene, then you will need antibiotics for selection in your plates.
The steps involved in molecular cloning using bacterial transformation are outlined in this graphic flowchart below.
Alternatively, you can watch this video for an overview.
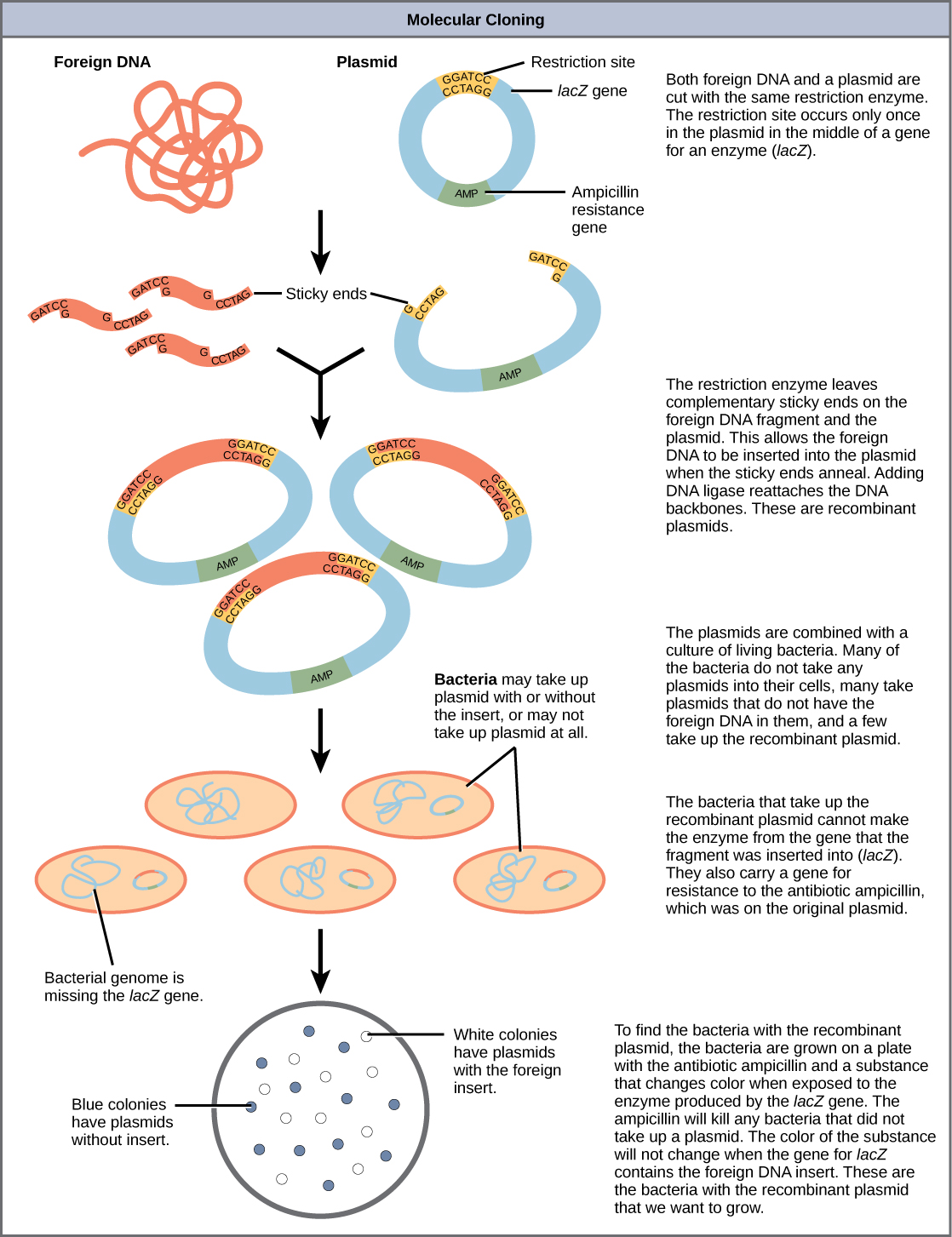
Cloning with Restriction Enzymes- Some Considerations
When designing a cloning experiment where you want to cut out an insert from one source such as genomic DNA and insert it into a second source such as a plasmid (vector), there are a few considerations.
1) The restriction sites should occur in the desired location in your recipient plasmid (usually in the Multiple Cloning Site (MCS) but not elsewhere in the vector!
2) They should be on either side of your insert, but do not cut within your insert!
3) Using 2 different enzymes makes cloning more efficient preventing the plasmid from reforming a circle without the inserted gene. This is also known as directional cloning.
Importantly it allows the inserted gene to be oriented in the correct position for transcribing the “sense” strand of DNA (the strand that codes for the protein)!
As we learned in Introduction to Transcription, the strand of DNA that has the coding sequence of the gene (5′-3′ direction) is called the Sense strand. The correct orientation in the recipient plasmid is key- you don’t want to express the antisense version of your gene!
See an example where I walk through the logic of picking restriction enzymes to use.
Cloning by PCR
While we mostly focused on restriction enzyme cloning. Another convenient way to isolate the DNA sequence of interest is Polymerase chain reaction (PCR)! The basic steps in PCR reaction were discussed earlier.
This is by far the most common method of cloning. In this method when designing primers for your region of interest researchers introduce a restriction site to the 5′ end of the primers.
Then upon amplification, the PCR product will have a restriction site added to either end that can be digested with the appropriate restriction enzyme. If the other primer has a different restriction sequence then the PCR fragment can be inserted in a directional-dependent manner in a host plasmid.
Types of Plasmids/Vectors
There are many different types of plasmids or vectors for use in molecular biology experiments. The choice of vector depends on the biological question or final use of the cloned DNA. Some types of vectors we will learn about are
General Purpose Vectors: Used to facilitate the cloning of DNA fragments. Cloning vectors tend to be very simple, often containing only a bacterial resistance gene, the origin of replication, and MCS. They are small and optimized to help in the initial cloning of a DNA fragment.
Genome Engineering Plasmids: Used for editing and modifying genes using CRISPR, which we shall see in the Genome Editing unit.
Expression Vectors: Used for gene expression. Expression vectors therefore contain additional sequences that will drive transcription and translation of the inserted gene! Therefore they must contain a promoter sequence, a transcription terminator sequence.
The promoter region is required for the generation of RNA from the inserted DNA via transcription. The terminator sequence on the newly synthesized RNA signals for the transcription process to stop.
Many sophisticated variations on such vectors have been created that have made it easy to produce and purify large amounts of any protein of interest for which the gene has been cloned.
For example: A handy feature in some expression vectors is a sequence encoding an affinity tag either up- or downstream of the gene being expressed. This sequence allows a short affinity tag (such as a run of histidine residues) to be fused onto the encoded protein. The tag can be used to readily purify the protein, as described in the section on affinity chromatography using special beads that bind to His-tag.
Another handy feature is fusing the coding sequence of fluorescent proteins like GFP to the coding sequence of a gene of interest and creating a recombinant fusion protein. This allows researchers to view the presence of the protein inside a living cell by microscopy. The two genes are under the same promoter element, transcribed as though it is one gene into a single messenger RNA molecule. The mRNA is then translated into protein.
To connect back to protein folding, in these cases, both proteins must be able to properly fold into their active conformations when fused and interact with their substrates despite being fused.
GFP protein is small and is a single-domain protein. It has been shown to adopt its three-dimensional shape independent of the addition of extra amino acids in many cases. Creating fluorescent fusion proteins is a very important tool used by cell biologists (see sidebar link to learning for stunning live cell microscopy of a 10-day tumor metastasizing)
To see a 10-day time-lapse of an engineered human micro-tumor forming and metastasizing click here.
More at: https://www.nikonsmallworld.com/galleries/2021-small-world-in-motion-competition
In all examples of Expression Cloning, where the goal is to transcribe mRNA and/or also have the host organism (Bacterial or Eukaryotic) make protein cloning; directionality matters!
The ‘sense’ strand of the DNA must be downstream of the promoter!
While recombinant proteins are often prepared in bacterial cells, it is not uncommon to express and purify recombinant proteins from Eukaryotic Cells! In this case, the expression vectors will contain the relevant sequences (for example eukaryotic promoter sequences ) that will direct expression inside eukaryotic cells.
Another special type of expression vector is a reporter plasmid which we discuss in detail below.
Reporter Plasmids and Reporter Gene Assays: Tool to Study Gene Expression
A common tool for studying the regulation of gene expression by cis- or trans-acting factors is reporter gene assays.
In these assays, a ‘reporter gene’ acts as a surrogate (reports) for the coding region of the gene under study.
Commonly used reporter genes are those that have visually identifiable characteristics and involve fluorescent or luminescent proteins.
Examples include the gene that encodes jellyfish green fluorescent protein (GFP), which causes cells that express it to glow green under blue light, and the enzyme luciferase, which catalyzes a reaction with luciferin to produce light.
Other genes that serve as good reporters are enzymes that upon the addition of a substrate generate a color that can be measured.
A common example of such a gene is Lac Z from E.coli which encodes β-galactosidase. This enzyme causes bacteria expressing the gene to appear blue when grown on a medium that contains the substrate analog X-gal or cells that express the gene to stain blue. The choice of the reporter gene will often depend on biological questions.
Using recombinant DNA technology one or more gene regulatory elements being analyzed are introduced (cloned) upstream of the coding sequence of the reporter gene. Following the introduction of the reporter construct into cells and experimental treatment, expression levels of the reporter gene are monitored by quantifying the reporter protein enzymatic activity.
References and Attributions
This chapter contains material taken from the following CC-licensed content. Changes include rewording, removing paragraphs replacing them with original material, and combining material from the sources.
1. Bergtrom, Gerald, “Cell and Molecular Biology 4e: What We Know and How We Found Out” (2020). Cell and Molecular Biology 4e: What We Know and How We Found Out – All Versions. 13.
https://dc.uwm.edu/biosci_facbooks_bergtrom/13
2. Works contributed to LibreTexts by Kevin Ahern and Indira Rajagopal. LibreTexts content is licensed by CC BY-NC-SA 3.0. The entire textbook is available for free from the authors at http://biochem.science.oregonstate.edu/content/biochemistry-free-and-easy
3. Genetics, Agriculture, and Biotechnology by Walter Suza; Donald Lee; Marjorie Hanneman; and Patricia Hain is licensed under a Creative Commons Attribution-NonCommercial-ShareAlike 4.0 International License
4. OpenStax Microbiology. Provided by: OpenStax CNX. Located at: http://cnx.org/contents/e42bd376-624b-4c0f-972f-e0c57998e765@4.2. License: CC BY: Attribution. License Terms: Download for free at http://cnx.org/contents/e42bd376-624b-4c0f-972f-e0c57998e765@4.2