1 Chapter 1. Earth’s Climate System
Introduction
Earth’s climate is a complex system with a number of key components – the atmosphere, the hydrosphere (oceans, lakes and rivers), the cryosphere (snow and ice), the geosphere (soils and rocks) and the biosphere (living things), all of which play important roles. Living animals and plants act as sources and sinks for carbon. Snow and ice are of paramount importance in controlling the planet’s albedo – the amount of sunlight that may be reflected straight back into space. The oceans act as sinks and sources for both carbon and heat energy and are sensitive to atmospheric conditions overhead.
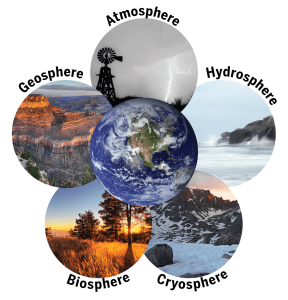
The Atmosphere
Though oxygen is crucial for life on Earth, it is not the primary component of our atmosphere. Earth’s atmosphere is composed of approximately 78 percent nitrogen, 21 percent oxygen, 0.93 percent Argon, 0.04 percent carbon dioxide as well as trace amounts of neon, helium, methane, krypton, ozone and hydrogen, as well as water vapor. In addition to gasses our atmosphere contains aerosols, solid natural particles (pollen, dust, volcanic ash, soot from forest fires) as well as solid particles released by human activities (smog, black carbon, soot).
Atmosphere is the mixture of gases that surrounds Earth’s surface, extending upwards for several hundred kilometers. It is divided up into several layers, the lowest of which is the Troposphere, which is where most weather occurs. Containing about 80% of the molecules that make up the atmosphere, it varies in thickness according to air temperature: thus in cold Polar regions it is less thick compared to the warm Tropics, where it can reach 17km in thickness. At its top – the Tropopause – there is a massive temperature inversion: whilst it gets colder with altitude in the troposphere, it suddenly warms up at the Tropopause.
Above the Tropopause is the Stratosphere, a thick layer of very thin air which extends up to about 50km above the surface. It contains about 20% of the molecules that make up the atmosphere: importantly these include a layer enriched in ozone, which blocks a lot (>90%) of harmful UV light from the Sun. Without that ozone, life as we know it would not exist as it does. At the top of the Stratosphere is the Stratopause, which marks the boundary with the very cold, extremely thin air Mesosphere. This is where most meteors burn up. Containing just 0.1% of the molecules in the atmosphere, it is a hostile place in which temperatures may be as low as –100oC near the top – the Mesopause. The Mesopause, only a few kilometers thick, marks the boundary with the Thermosphere above, which is a deep (several hundred km), virtually airless layer, where satellites as well as the International Space Station orbit the planet.

Constantly on the move – just as the oceans – the air making up the atmosphere transports heat, cold, moisture and clouds over the planet’s surface. Major frontal zones between quasi-permanent warm and cold air masses are marked by jet streams along which active weather-systems develop. Heat and moisture are exchanged with all other components of the climate system. Warmer air carries more water-vapor available to condense and fall as rain; rain transfers heat directly to land, rivers and oceans. Conversely, direct solar heating of land in favorable conditions causes warmed air to rise swiftly upwards, carrying with it moisture, to cause convective thunderstorms.
Clouds reflect incoming solar radiation from their white tops but conversely act as insulating blankets trapping in heat beneath them. Greenhouse gases (especially carbon-dioxide and water-vapor) impede the escape of space-bound infra-red heat energy: without them, Earth would be a frozen ball of ice at its distance from the Sun. However, the steady increase in their concentrations due to the burning of the fossil fuels has caused what on human life scales may appear to be a gradual increase in the global energy imbalance, but which is, in geological terms, extremely steep.
The Cryosphere
The snow and ice on planet Earth consists of five subsets: the ice-sheets, polar sea-ice, permafrost, mountain glaciers and seasonal snow-cover. Its biggest part is the large ice-sheets that sit atop Greenland and the Antarctic. These have both been losing mass in recent decades due to an acceleration of melting. Such melting makes a direct contribution to sea-level rise.
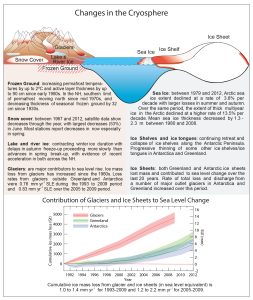
Next most important (in terms of size) is sea-ice, which because of its large changes in surface area during its seasonal melting and regrowing seasons is an important variable in terms of Earth’s albedo. Sea-ice melt has no bearing on sea-level changes. However, increased seasonal melt opens more water to receiving and absorbing incoming solar radiation. In the Arctic, sea-ice area, extent and volume have all exhibited a significant downward trend. In the Antarctic, sea-ice has exhibited a weak upward trend, more as a function of changes to regional wind-patterns than any imagined cooling. It is important to note that the Arctic and Antarctic are geographical opposites in more than one way: the Arctic is an ocean surrounded by landmasses, whereas the Antarctic is a huge landmass surrounded by ocean. This difference has a considerable bearing on the factors that influence seasonal sea-ice behavior.
The third subset is permafrost – permanently-frozen ground. Permafrost is important because it locks up a lot of trapped greenhouse gases like carbon dioxide and methane. However, it is becoming increasingly unstable in many areas: regionally-enhanced warming, such as that occurring in the Arctic and adjacent areas, is leading to permafrost melt, with accompanying release of the gases once they are able to make their way to the surface. The quantities of such gases at risk of release due to permafrost-melt are very substantial indeed.
Mountain glaciers are of prime climatological importance in terms of water-storage. Himalayan glaciers are particularly vital in such terms, providing a reliable source of river-water to the plains below. Mountain glaciers continue to retreat around the world, except for areas where the supply of what ends up as glacial ice – snow – is increasing to the extent that ice supply outdoes ice-loss.
Seasonal snow-cover and its extent/duration are important with respect to albedo, and the lesser its extent/shorter its duration, the longer the period during which the rocks/soils on which it falls can receive incoming solar radiation. The trend in both of these variables is downwards.
The Geosphere
The land surface of the planet acts as a sink for incoming short-wave solar energy and it re-radiates infra-red radiation (IR) as it warms up. Albedo is important here too: darker rocks absorb more solar energy and emit more IR – that’s why black tarmac feels hot on a sunny summer’s day. Land is also an important carbon sink: carbon dioxide dissolved in rainwater reacts chemically with some of the minerals making up the rocks. Topography is important: mountain ranges force air up over them, cooling it as it rises and enhancing rainfall. Conversely, because of that enhanced rainfall, areas downwind of mountains receive dryer air and tend to experience drier climates.
Plate tectonics demonstrates how, like other aspects of the climate system, the geosphere is constantly on the move. However, compared to the other Earth Climate System components, the movement is very slow indeed. The geosphere has periodic perturbations that may drastically effect the overall climate in the short term, such as volcanic eruptions. Volcanoes are one important non-manmade source of carbon dioxide, emitting anything up to 300 million tons of the gas per year. This sounds like a lot but when placed against manmade emissions – 30,000 million tons a year is our current contribution – it falls by the wayside. Nevertheless, further back in geological time there have been eruptions that were off-the-scale compared to anything in human history and these likely did have significant effects on the climate.
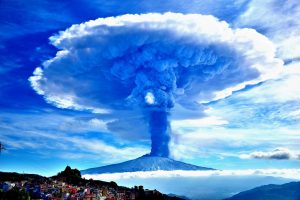
Major eruptions can, conversely, lead to cooling effects in the atmosphere due to the amount of dust and volcanic aerosols such as sulphur compounds that are blasted kilometers up into the air. Although sometimes severe in nature, such effects tend to be short-lived because gravity and rainfall bring most of the dust and aerosols back to the planet’s surface within just a few years. By contrast, the greenhouse gas carbon-dioxide stays in the atmosphere much longer, hundred years or so. The reason is because the fastest carbon-dioxide sink – plant life – is also carbon-neutral; plants take it in but re-release it when they die and decompose, which is an effectively continual process, recycling a lot of carbon dioxide all the time. That leaves other major carbon dioxide sinks such as weathering of rocks and absorption by the oceans, which are much slower processes for a number of reasons. That slowness effectively creates a bottleneck in the system, which means that if we add the gas to the atmosphere faster than it can be taken back in then levels will go up, as they have done.
The Hydrosphere
The oceans are where most of Earth’s liquid water occurs and they constitute about 71% of Earth’s surface. They are an important sink for carbon: they both absorb it directly from the atmosphere and indirectly via the weathering of rocks. Dissolved carbon in the shallower waters is re-precipitated as calcium carbonate, forming sea-shells, the skeletons of corals and other invertebrates. In deeper water, calcium carbonate becomes more soluble, and beyond a certain depth it dissolves quicker than it forms (the carbonate compensation depth).
Sea-water is weakly alkaline: on the pH scale of 0 (extremely acid) to 14 (extremely alkaline), with neutral being 7, it has a pH of just over 8. However, dissolution of excessive carbon dioxide in seawater interferes with its chemistry by reducing the pH. It is important to understand that only small such changes, if rapid in nature, can lead to major problems in marine ecosystems. This is simply because marine organisms that build their shells or exoskeletons from calcium carbonate that they obtain from seawater are adapted to do so in a relatively narrow pH range. Stray outside of that range and their ability to build is severely diminished. Such organisms form the foundations of marine food-chains; if their existence is compromised then so is the existence of a spectrum of higher predators, including fish. This is why ocean acidification, as it’s called, is an issue that requires urgent attention.
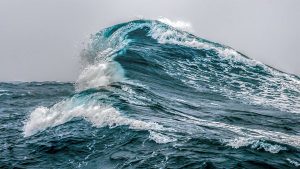
The oceans, constantly moving due to currents, winds and tides, also absorb and transport heat around the planet: over 90% of the total global warming effect is taking place within them. Surface warming occurs via incoming solar radiation and from addition of warm water from rivers, sometimes an important factor, for example in the Russian Arctic where continent-crossing rivers transport heat northwards. As oceanic water warms it expands, thereby contributing to rising sea-levels.
In terms of heat energy, the oceans have long been regarded as having three layers: an upper one down to 700m, a lower one from 700-2000m and, beneath 2000m, the cold abyssal waters. The transport of heat energy between the upper and lower layers is a major factor in climate change. Vertical mixing of ocean-water can occur due to currents, downwelling and upwelling, storms/prevailing winds and, more locally, tides.
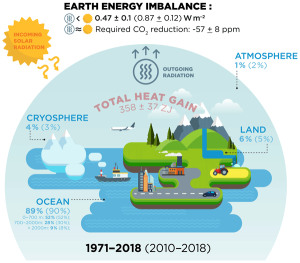
In some areas, particularly in the Southern Hemisphere, fluctuations in persistent weather-patterns lead to prolonged periods at times in which the surface waters of the upper layer are acting as an especially strong heat source or, alternatively, a heat sink. Thus, in the best-known example, in the Pacific Ocean, they can cause a strong atmospheric warming (El Nino) or cooling (La Nina) effect over periods of a year or more. These fluctuations are expressed numerically by the monthly Oceanic Nino Index. Over short timespans, the effects of such fluctuations are far greater than the long-term, multidecadal trend due to manmade greenhouse gas emissions, which is why the surface air temperature records have such a noisy appearance with lots of peaks and troughs.
The Biosphere
An important source and sink of greenhouse gases, especially carbon-dioxide, water vapor and methane, the biosphere is the living bit of the planet and comprises all ecosystems from soils to forests, from coral-reefs to plankton-rich shallow waters. It also includes humanity, of course, and its industrial/resource-consuming activities. The biosphere has little role as a direct heat source or sink, but indirect effects due to its massive influences on greenhouse gas levels are of prime importance. Not for nothing have the world’s forests – especially the great Amazon rain-forest – been called ‘the lungs of the planet’. This is all down to photosynthesis – the absorption by green plants of carbon-dioxide and their excretion of oxygen as a metabolic waste-product. Photosynthetic plants – from the lowliest blue-green algae up to the mightiest trees – are responsible for our planet’s oxygenated atmosphere. The evolution of cyanobacteria (stromatolites) about 3.7 billion years ago and their photosynthesis released oxygen which immediately oxidized soluble iron in sea-water getting to insoluble iron oxides, also known as BIFs (Banded Iron Formations). Once free oxygen began to accumulate in the atmosphere, the transition towards the composition of the air we breathe today was well underway.
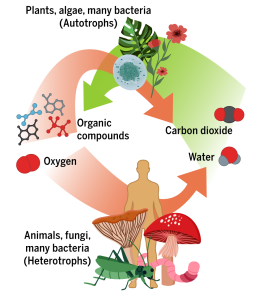
The early history of biosphere is enough to demonstrate clearly how vital a healthy biosphere is for Mankind and that it should not simply be regarded as a resource that can be destroyed in the name of profit. Without the evolution of photosynthetic plant life, none of us would be here today. Like other mammals, we are an integral part of the biosphere and we utterly rely on it for our existence. However, there are few points along the geological timescale where life itself has significantly altered the composition of Earth’s atmosphere. One was the aforementioned oxygenation event. Another occurred during the Devonian and Carboniferous periods, 419-299 million years ago, a time during which land was firstly colonized by an abundance of diverse plant life. This caused a major drawdown of atmospheric carbon dioxide, the levels of which up to that point were much greater. The third such episode is now underway: Mankind is digging up or pumping out the fossilized products (coal and oil) of that plant life and burning it as an energy-source, thereby returning a lot of that carbon dioxide back to the atmosphere.
Carbon Cycle
Forged in the heart of aging stars, carbon is the fourth most abundant element in the Universe. Most of Earth’s carbon—about 65,500 billion metric tons—is stored in rocks. The rest is in the ocean, atmosphere, plants, soil, and fossil fuels. Carbon flows between each reservoir in an exchange called the carbon cycle, which has slow and fast components. Any change in the cycle that shifts carbon out of one reservoir puts more carbon in the other reservoirs. Changes that put carbon gases into the atmosphere result in warmer temperatures on Earth.
Carbon moves from one storage reservoir to another through a variety of mechanisms. For example, in the food chain, plants move carbon from the atmosphere into the biosphere through photosynthesis. They use energy from the sun to chemically combine carbon dioxide with hydrogen and oxygen from water to create sugar molecules. Animals that eat plants digest the sugar molecules to get energy for their bodies. Respiration, excretion, and decomposition release the carbon back into the atmosphere or soil, continuing the cycle.
The ocean plays a critical role in carbon storage, as it holds about 50 times more carbon than the atmosphere. Two-way carbon exchange can occur quickly between the ocean’s surface waters and the atmosphere, but carbon may be stored for centuries at the deepest ocean depths.
Rocks like limestone and fossil fuels like coal and oil are storage reservoirs that contain carbon from plants and animals that lived millions of years ago. When these organisms died, slow geologic processes trapped their carbon and transformed it into these natural resources. Processes such as erosion release this carbon back into the atmosphere very slowly, while volcanic activity can release it very quickly. Burning fossil fuels in cars or power plants is another way this carbon can be released into the atmospheric reservoir quickly.
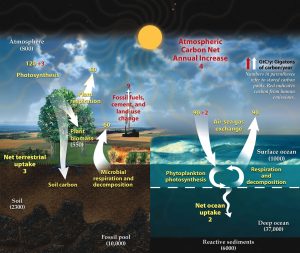
The time it takes carbon to move through the fast carbon cycle is measured in a lifespan. The fast carbon cycle is largely the movement of carbon through life forms on Earth, or the biosphere. Human activities have a tremendous impact on the fast carbon cycle. Burning fossil fuels, changing land use, and using limestone to make concrete, all transfer significant quantities of carbon into the atmosphere. As a result, the amount of carbon dioxide in the atmosphere is rapidly rising; it is already considerably greater than at any time in the last 800,000 years. The ocean absorbs much of the carbon dioxide that is released from burning fossil fuels. This extra carbon dioxide is lowering the ocean’s pH, through a process called ocean acidification. Ocean acidification interferes with the ability of marine organisms to build their shells and skeletons.
Conclusions
All components of the climate system are affected by the increase in the energy imbalance caused by rising greenhouse gas concentrations resulting from burning fossil fuels: rising atmospheric and oceanic temperatures, reductions in polar and glacial ice, thaw of ancient permafrost, migrations of species as their favored climatic zones move poleward, all being examples with a common cause. Therefore, it is unwise to just look at surface air temperatures when discussing climate change: they are just a part of the multi-component system that is Earth’s warming climate.
This Chapter is compiled from multiple resources including: NASA’s Ocean Motion and Sea Currents, NASA’s Global Climate Change, NOAA’s National Centers for Environmental Information, NOAA’s Climate gov., Intergovernmental Panel on Climate Change, and Nature Communications.