6 Chapter 6. Human and Ecosystem Vulnerabilities due to Climate Change
Sea Level Rising
Sea level has been rising over the past century, and the rate has increased in recent decades. In 2017, global mean sea level was 7.7 cm (3 inches) above the 1993 average—the highest annual average in the satellite record (1993-present). It was the sixth consecutive year, and the 22nd out of the last 24 years in which global mean sea level increased relative to the previous year. The pace of global sea level rise nearly doubled from 1.7 mm (1/15 in) per year throughout most of the twentieth century to 3.1 mm (1/8 inch) per year since 1993. The rising sea level is mostly due to a combination of meltwater from glaciers and ice sheets and thermal expansion of seawater as it warms.
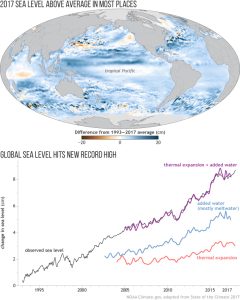
Figure 1 Details.
Top: Global map showing where sea level in 2017 was higher (blue) or lower (brown) than the 1993-2017 average. Bottom: Diagram showing rising sea level from 1993 to 2017 and contribution of glacier melting and warm water expansion to it. (Source: NOAA Climate.gov map)
Globally, 8 of the world’s 10 largest cities are near a coast, according to the U.N. Atlas of the Oceans. In the United States, almost 40 percent of the population lives in relatively high population-density coastal areas, where sea level plays a role in flooding, shoreline erosion, and hazards from storms. In urban settings along coastlines rising seas threaten infrastructure necessary for local jobs and regional industries. Roads, bridges, subways, water supplies, oil and gas wells, power plants, sewage treatment plants, landfills—the list is practically endless—are all at risk from sea level rise. Higher sea levels mean that deadly and destructive storm surges – like those associated with Hurricane Katrina or “Superstorm” Sandy – push farther inland than they once did. Rising sea level creates stress on coastal ecosystems that provide recreation, protection from storms, and habitat for fish and wildlife, including commercially valuable fisheries. As seas rise, saltwater is also intruding into freshwater aquifers, many of which sustain municipal and agricultural water supplies and natural ecosystems.
What’s causing sea level to rise?
Sea level is rising for two main reasons: glaciers and ice sheets are melting and adding water to the ocean and the volume of the ocean is expanding as the ocean water warms. A third, much smaller contributor to sea level rise is a decline in water storage on land—aquifers, lakes and reservoirs, rivers, soil moisture—mostly as a result of groundwater pumping, which has shifted water from aquifers to the ocean.
From the 1970s up through the last decade, melting and thermal expansion were contributing roughly equally to the observed sea level rise. But the melting of glaciers and ice sheets has accelerated, and over the past decade, the amount of sea level rise due to melting—with a small addition from groundwater transfer and other water storage shifts—has been nearly twice the amount of sea level rise due to thermal expansion.
Glacier mass loss accelerated from 226 gigatons per year between 1971 and 2009 to 275 gigatons per year between 1993 and 2009. Ice loss from the Greenland Ice Sheet increased six-fold, from 34 gigatons per year between 1992-2001 to 215 gigatons per year between 2002 and 2011. Antarctic ice loss more than quadrupled, from 30 gigatons per year between 1992 and 2001 to 147 gigatons per year from 2002 to 2011.
Future sea level rise
As global temperatures continue to warm, sea level will continue to rise. How much it will rise depends mostly on the rate of future carbon dioxide emissions and future global warming. How fast it will rise depends mostly on the rate of glacier and ice sheet melting.
The pace of sea level rise accelerated beginning in the 1990s, coinciding with acceleration in glacier and ice sheet melting. However, it’s uncertain whether that acceleration will continue, driving faster and faster sea level rise, or whether internal glacier and ice sheet dynamics will lead to “pulses” of accelerated melting interrupted by slowdowns.
In 2012, at the request of the U.S. Climate Change Science Program, NOAA scientists conducted a review of the research on global sea level rise projections, and concluded that there is very high confidence (greater than 90% chance) that global mean sea level will rise at least 20 cm (8 inches) but no more than 2 meters (6.6 feet) by 2100.
Vulnerability of U.S. coasts due to sea level rise
America’s trillion-dollar coastal property market and public infrastructure are threatened by the ongoing increase in the frequency, depth, and extent of tidal flooding due to sea level rise, with cascading impacts to the larger economy. Higher storm surges due to sea level rise and the increased probability of heavy precipitation events exacerbate the risk. Under a higher scenario Representative Concentration Pathway (RCP8.5), many coastal communities will be transformed by the latter part of this century, and even under lower scenarios (RCP4.5 or RCP2.6), many individuals and communities will suffer financial impacts as chronic high tide flooding leads to higher costs and lower property values. Actions to plan for and adapt to more frequent, widespread, and severe coastal flooding would decrease direct losses and cascading economic impacts. [A Representative Concentration Pathway (RCP) is a greenhouse gas concentration trajectory adopted by the IPCC. Four pathways were used for climate modeling and research for the IPCC fifth Assessment Report (AR5) in 2014. The pathways describe different climate futures, all of which are considered possible depending on the volume of greenhouse gases (GHG) emitted in the years to come. The RCPs – originally RCP2.6, RCP4.5, RCP6, and RCP8.5 – are labelled after a possible range of radiative forcing values in the year 2100 (2.6, 4.5, 6, and 8.5 W/m2, respectively)]
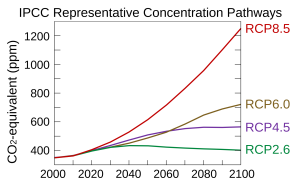
Fisheries, tourism, human health, and public safety depend on healthy coastal ecosystems that are being transformed, degraded, or lost due in part to climate change impacts, particularly sea level rise and higher numbers of extreme weather events. Restoring and conserving coastal ecosystems and adopting natural and nature-based infrastructure solutions can enhance community and ecosystem resilience to climate change, help to ensure their health and vitality, and decrease both direct and indirect impacts of climate change.
As the pace and extent of coastal flooding and erosion accelerate, climate change impacts along our coasts are exacerbating preexisting social inequities, as communities face difficult questions about determining who will pay for current impacts and future adaptation and mitigation strategies and if, how, or when to relocate. In response to actual or projected climate change losses and damages, coastal communities will be among the first in the Nation to test existing climate-relevant legal frameworks and policies against these impacts and, thus, will establish precedents that will affect both coastal and non-coastal regions.
Loss of Ice in Antarctica and Greenland
The Antarctic Ice Sheet covers nearly 14 million square kilometers (5.4 million square miles). The Greenland Ice Sheet covers roughly 1.7 million square kilometers (650,000 square miles). Together, the Greenland and Antarctic ice sheets hold about 99 percent of the world’s freshwater ice. If the Greenland Ice Sheet melted away completely, sea level would rise roughly 7 meters, or 23 feet (Gregory et al. 2004). If the entire Antarctic Ice Sheet melted, sea level would rise by about 57 meters, or 187 feet (Lythe et al. 2001). While this is unlikely for the foreseeable future, even a partial loss of these huge ice masses could have a significant effect on coastal areas.
A key area of glaciological study in recent years is ice sheet mass balance. The mass balance of an ice sheet is the difference between its total snow input and the total loss through melting, ablation, or calving. So long as an ice sheet gains an equal mass through snowfall as it loses through melt, ablation, and calving from glaciers and ice shelves, it is said to be in balance. Because ice sheets contain so much ice and have the potential to raise or lower global sea level so dramatically, measuring the mass balance of the ice sheets and tracking any mass balance changes and their causes is very important for forecasting sea level rise.
Data from NASA’s GRACE satellites show that the ice sheets in both Antarctica and Greenland have been losing mass since 2002. Both ice sheets have seen an acceleration of ice mass loss since 2009.
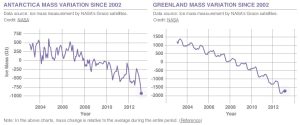
Figure 3 Details.
Data from NASA’s GRACE satellites show that the land ice sheets in both Antarctica and Greenland are losing mass. The continent of Antarctica (left chart) has been losing more than 100 cubic kilometers (24 cubic miles) of ice per year since 2002. (Source: NASA)
In 2009, using ICESat (Ice, Cloud, and land Elevation Satellite is the benchmark Earth Observing System mission for measuring ice sheet mass balance), measurements of both Greenland and Antarctica found that dynamic thinning (ice loss resulting from accelerated glacier flow) now reached all latitudes in Greenland, and had intensified at key areas of Antarctica’s grounding line. The study concluded that dynamic thinning lasts for decades after an ice shelf collapse, a situation that occurred several times in the late 1990s and early 2000s. Moreover, the thinning reached far inland. In other regions, warm sub-surface ocean water was shown to be responsible for thinning glaciers as they went afloat resulting in rapid acceleration of a broad area of the glacier. ICESat data indicated that basal melting was also thinning floating ice shelves, reducing their ability to buttress the glaciers feeding them. Because Antarctica drains more than 80 percent of its ice sheet through floating ice shelves, accelerated glacier flow has the potential to affect ice sheet mass balance dramatically and raise sea level.
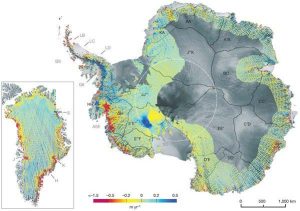
Figure 4 Details.
The rates of change in Greenland and Antarctica from 2003 to 2007. Dark blue indicates an increase of 0.5 meters per year, and dark red indicates a decrease of 1.5 meters per year. (Source: Pritchard et al. 2009)
The European Space Agency’s CryoSat-2 mission has enhanced Antarctic ice sheet monitoring by including areas closer to the poles than earlier satellites, and by acquiring better data in moderately sloping areas, including ice sheet margins where most of the ice loss occurs. CryoSat-2 observations taken between November 2010 and September 2013 indicate annual ice sheet mass losses of 134 ± 27 gigatonnes in West Antarctica, 3 ± 36 gigatonnes in East Antarctica, and 23 ± 18 gigatonnes on the Antarctic Peninsula. The Amundsen Sea showed the largest signal of ice loss.
Surface melt on an ice sheet not only directly reduces the ice sheet mass but also can accelerate ice flow and even leads to further melting. Surface meltwater can penetrate through cracks in the surface, and force them open, allowing large amounts of water to drain to the bed and spread out across the base of the ice sheet, lubricating it.
In February and March of 2002, the Larsen B Ice Shelf on the Antarctic Peninsula underwent rapid disintegration. Warm summertime temperatures led to the formation of melt ponds on the ice surface. Some of this meltwater infiltrated cracks in the ice, slicing through the shelf. The increased amount of fracturing, and possibly changes at the ice shelf margins (loss of connection with the coastline) and wave action (flexing the shelf a slight amount), led to the break-up of the shelf. Similar events have occurred before (Larsen A Ice Shelf in 1995, Larsen Inlet Ice Shelf in 1986 or 1987) and since (northwestern Wilkins Ice Shelf in 2008), but so far these events are limited to the Antarctic Peninsula. The Peninsula is the fastest-warming part of the continent.
Arctic and mountain glaciers
The impacts of climate change are being observed earlier in the Arctic, and with more immediate and severe consequences than in most of the rest of the world. The Arctic is warming at a rate almost twice the global average and reductions in Arctic sea-ice and permafrost and changes in weather are increasingly visible. The effects of a warming atmosphere on physical, chemical, biological, and human components of Arctic ecosystems are myriad, far-reaching, and accelerating. The warming has caused a cascade of physical changes, from direct effects such as the melting of sea-ice and sea level rise, to secondary effects such as decreased albedo (surface reflectivity) and coastal erosion, to tertiary effects such as the accelerated warming of the ocean due to feedback loops between different climate factors.
As warming in the Arctic has progressed, sea-ice has changed in both quantity (reduction in ice extent and volume) and quality (sea-ice fragmentation, deterioration, and altered seasonality), with profound consequences for sea-ice dependent species. Such consequences may be in the form of both reduced fitness of individual animals and altered population parameters. Many Arctic marine mammals will also be affected indirectly as the food webs on which they depend undergo changes. Restructured food webs, changes in prey populations and the arrival of new marine mammal species (including new predators), competitors, and pathogens from more temperate seas will challenge the Arctic species.
Declines in the amount and thickness of sea ice are creating more opportunities for human activity in the Arctic, with resulting impacts on marine mammals. The earlier disappearance of sea ice from coastlines coupled with the ice’s retreat farther from shore during the summer and tendency to remain offshore longer in the fall means there is now a large, growing seasonal window of open water. This creates opportunities for oil and gas exploration and development, shipping, tourism, commercial fishing, and military operations. These activities expose Arctic marine mammals to a variety of threats, including ship strikes, pollution, entanglement in fishing nets or lines, and exposure to human-caused sound and other forms of disturbance that previously either had been absent or had been present only on a much smaller scale.
If the potentially catastrophic impact of climate change on some Arctic species is to be avoided, the source of the problem on a global scale will have to be addressed. Regardless of the degree to which society and governments are able to alter the course of global warming, our challenge in the short-term is to understand the effects of climate change on the Arctic, and anticipate the impact on Arctic species and human communities to the fullest extent possible.
From roughly 1300 to 1850, glaciers in the European Alps were substantially bigger than they are today, a defining characteristic of the Little Ice Age. Around 1865, Alpine glaciers began retreating. In general, glaciers retreat due to a combination of rising temperatures and declining precipitation, but the temperature and precipitation records from the Alps indicated that glaciers should have continued advancing until around 1910. A more plausible explanation for the mid-19th-century retreat involves black carbon. Black-carbon emissions rose significantly after the mid-19th century, and didn’t abate until well into the 20th century. By lowering albedo, soot from the fossil fuel power generating plants and factories raised the amount of solar radiation absorbed by snow, leading to Alpine glacier retreat. Glacier retreat starting in the mid-19th century was not a global phenomenon; glaciers in Argentina did not begin retreating until the early 20th century.
New research (Marzeion et al. 2014) indicated that, starting in the late 1970s, mountain glaciers “crossed an invisible line” into a declining state that cannot easily be attributed to natural causes. From 1851 to 2010 only 35 percent of the glacier mass loss could be attributed to anthropogenic causes, but from 1991 to 2010, the anthropogenically caused glacier mass loss increased to 69 percent. The study found that the anthropogenic signal from 1991 to 2010 was detectable with high confidence.
Ocean water and life
Global sea levels may rise by as much as 69 cm during the next 100 years due to melting of glaciers and polar ice, and thermal expansion of warmer water. Rising water levels will have serious impacts on marine ecosystems. The amount of light reaching offshore plants and algae dependent on photosynthesis could be reduced, while coastal habitats are already being flooded. Rapid sea level rise will likely be the greatest climate change challenge to mangrove ecosystems, which require stable sea levels for long-term survival.
Vertical mixing in the ocean is important for many reasons, including transporting nutrients from deep to shallow waters, and surface water rich in oxygen into deeper waters. In some areas, changes to ocean temperature profiles induced by climate change are causing a reduction in the amount of mixing and for reducing oxygen levels at depth.
After absorbing a large proportion of the carbon dioxide released by human activities, the oceans are becoming acidic. If it weren’t for the oceans, the level of carbon dioxide in the atmosphere would be much higher. The effect could be that fish, squid, and other gilled marine animals may find it harder to “breathe”, as the dissolved oxygen essential for their life becomes difficult to extract as water becomes more acidic. Also, shellfish, crabs, lobsters, and corals may find it more difficult to build their calcium carbonate shells. In some areas, calcium carbonate shells may even start to dissolve.
Most scientists believe that global warming will herald a new era of extreme and unpredictable weather. Tropical storms and heavier rainfall may increase and so too would the consequent physical damage to coral reefs, other coastal ecosystems, and coastal communities.
The marine environment is already registering the impacts of climate change. The current increase in global temperature of 0.7°C since pre-industrial times is disrupting life in the oceans, from the tropics to the poles. Marine species affected by climate change include plankton – which forms the basis of marine food chains – corals, fish, polar bears, walruses, seals, sea lions, penguins, and seabirds. The Intergovernmental Panel on Climate Change predicts a further rise of between 1.4°C and 5.8°C by the end of the century. Climate change could therefore well be the knock-out punch for many species which are already under stress from overfishing and habitat loss.
One of the most visually dramatic effects of climate change is coral bleaching, a stress response caused by high water temperatures that can lead to coral death. Recent years have seen widespread and severe coral bleaching episodes around the world, with coral mortality reaching 70% in some regions.
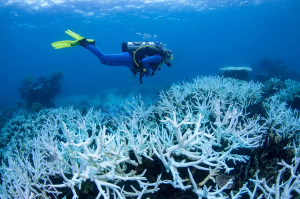
Figure 5 Details.
Australia’s Great Barrier Reef has experienced four mass bleaching events in the last seven years, like this one in 2017. Repeated bleaching makes it tough for corals to recover. (Photo: B.M. Garner)
As the oceans warm, the location of the ideal water temperature may shift for many species. A study has shown that fish in the North Sea have moved further north or into deeper water in response to rising sea temperatures. The ranges of many fish and shellfish species may change. In waters off the northeastern United States, several economically important species have shifted northward since the late 1960s. The three species, American lobster, red hake, and black sea bass, have moved northward by an average of 119 miles. Other species may lose their homes for other reasons. The distribution of penguin species in the Antarctic Peninsula region, for example, is changing with reductions in sea ice due to global warming.
Rising temperatures can directly affect the metabolism, life cycle, and behavior of marine species. For many species, temperature serves as a cue for reproduction. Clearly, changes in sea temperature could affect their successful breeding. The number of male and female offspring is determined by temperature for marine turtles, as well as some fish and copepods (tiny shrimp-like animals on which many other marine animals feed). Changing climate could therefore skew sex ratios and threaten population survival.
Agriculture and Food Supply
Moderate warming and more carbon dioxide in the atmosphere may help some plants to grow faster. However, more severe warming, floods, and drought may reduce yields. Livestock may be at risk, both directly from heat stress and indirectly from reduced quality of their food supply. Fisheries will be affected by changes in water temperature that make waters more hospitable to invasive species and shift the ranges or lifecycle timing of certain fish species.
Climate change is very likely to affect food security at the global, regional, and local level. Climate change can disrupt food availability, reduce access to food, and affect food quality. For example, projected increases in temperatures, changes in precipitation patterns, changes in extreme weather events, and reductions in water availability may all result in reduced agricultural productivity. Increases in the frequency and severity extreme weather events can also interrupt food delivery, and resulting spikes in food prices after extreme events are expected to be more frequent in the future. Increasing temperatures can contribute to spoilage and contamination.
Internationally, these effects of climate change on agriculture and food supply are likely to be similar to those seen in the United States. However, other stressors such as population growth may magnify the effects of climate change on food security. In developing countries, adaptation options like changes in crop-management or ranching practices, or improvements to irrigation are more limited than in the United States and other industrialized nations.
Any climate-related disturbance to food distribution and transport, internationally or domestically, may have significant impacts not only on safety and quality but also on food access. For example, the food transportation system in the United States frequently moves large volumes of grain by water. In the case of an extreme weather event affecting a waterway, there are few, if any, alternate pathways for transport. High temperatures and a shortage of rain in the summer of 2012 led to one of the most severe summer droughts the nation has seen and posed serious impacts to the Mississippi River watershed, a major transcontinental shipping route for Midwestern agriculture.
This drought resulted in significant food and economic losses due to reductions in barge traffic, the volume of goods carried, and the number of Americans employed by the tugboat industry. The 2012 drought was immediately followed by flooding throughout the Mississippi in the spring of 2013, which also resulted in disruptions of barge traffic and food transport. Transportation changes such as these reduce the ability of farmers to export their grains to international markets, and can affect global food prices.
Infectious and vector borne diseases
Current evidence suggests that inter-annual and inter-decadal climate variability have a direct influence on the epidemiology of vector-borne diseases (Vector-Borne Disease is disease that results from an infection transmitted to humans and other animals by blood-feeding anthropods, such as mosquitoes, ticks, and fleas). By 2100 it is estimated that average global temperatures will have risen by 1.0–3.5oC, increasing the likelihood of many vector-borne diseases in new areas. Malaria and dengue fever are among the most important vector-borne diseases in the tropics and subtropics; Lyme disease is the most common vector-borne disease in the USA and Europe. Encephalitis is also becoming a public health concern. Health risks due to climatic changes will differ between countries that have developed health infrastructures and those that do not.
Human settlement patterns in the different regions will influence disease trends. While 70% of the population in South America is urbanized, the proportion in sub-Saharan Africa is less than 45%. Climatic anomalies associated with the El Niño–Southern Oscillation phenomenon and resulting in drought and floods are expected to increase in frequency and intensity. They have been linked to outbreaks of malaria in Africa, Asia and South America. Climate change has far-reaching consequences and touches on all life-support systems. It is therefore a factor that should be placed high among those that affect human health and survival.
The tropical African climate is favorable to most major vector-borne diseases, including: malaria, schistosomiasis, onchocerciasis, trypanosomiasis, filariasis, leishmaniasis, plague, Rift Valley fever, yellow fever and tick-borne hemorrhagic fevers. The continent has a high diversity of vector-species complexes that have the potential to redistribute themselves to new climate-driven habitats leading to new disease patterns. These organisms have different sensitivities to temperature and precipitation.
Vector species in Africa have adapted to ecosystems ranging from humid forests to dry savannas. As these ecosystems change so will the distribution of vectors species. Factors that alter the resting sites for adult tsetse flies, such as long-term changes in rainfall, can affect the epidemiology and transmission of trypanosomiasis. Extreme weather events that cause flooding will intensify the transmission of desert malaria and Rift Valley fever.
The most important climate-sensitive vector-borne diseases in South America as far as the numbers of people affected are concerned are malaria, leishmaniasis, dengue fever, Chagas disease and schistosomiasis. Other vector-borne diseases with relatively low number of cases occurring each year, and which may be sensitive to climate shifts, are yellow fever, plague, Venezuelan equine encephalitis, and other arboviral infections. Studies have shown that unusually dry conditions — for example, those caused by weather related to the El Niño–Southern Oscillation phenomenon in the northern part of the continent – are accompanied or followed by increases in the incidence of the disease. Recent estimates based on the Hadley Centre’s coupled atmosphere–ocean general circulation model projected that the additional number of people at risk of infection due to year round transmission of malaria in South America will rise from 25 million by year 2020 to 50 million by 2080.
The most important vector-borne diseases in Europe and some of the countries of the former Soviet Union are malaria and Lyme disease, which are transmitted by mosquitoes and ticks, respectively. Occasional outbreaks of malaria in Europe arise when infective mosquitoes are imported from the tropics by aircraft. A far greater problem is the growing number of patients who contract malaria overseas. This is particularly worrying because of the rapid spread of multidrug-resistant strains of the parasite; the occurrence of cases of untreatable malaria remains a distinct possibility.
As the climate warms, many vectors — not just those that transmit malaria — are likely to expand their ranges within Europe and new vector species may be introduced from the tropics. Tick distributions are also closely linked with climate, and there is growing concern that tick-borne diseases, such as Lyme disease and tick-borne encephalitis, may be increasing in northern Europe. Leishmaniasis is endemic in many parts of southern Europe and is an important co-infection with human immunodeficiency virus (HIV). As the climate becomes warmer, the sandfly vectors of leishmaniasis may become more abundant, spreading north. Long hot summers are also ideal for other flies and the possibility exists of increasing cases of diarrhea transmitted by houseflies, Musca domestica, and other species of synanthropic flies.
In north-east Punjab, malaria epidemics increase fivefold in the year following an El Niño event, while in Sri Lanka the risk of malaria epidemics increases fourfold during an El Niño year. In Punjab, epidemics are associated with above-normal precipitation, and in Sri Lanka, with below-normal precipitation. In Asia, dengue fever and malaria have been associated with positive temperature and rainfall anomalies, while in Australia arboviral disease outbreaks are most frequently associated with flooding.
Lyme disease is a tick-borne bacterial disease that is endemic in parts of North America, Europe, and Asia. In the United States, Lyme disease is caused by the bacterium Borrelia burgdorferi sensu stricto and is the most commonly reported vector-borne illness. It is primarily transmitted to humans in the eastern United States by the tick species Ixodes scapularis known as blacklegged ticks or deer ticks, and in the far western United States by Ixodes pacificus, commonly known as western blacklegged ticks.
Illness in humans typically presents with fever, headache, fatigue, and a characteristic skin rash called erythema migrans. If left untreated, infection can spread to joints, the heart, and the nervous system. Since 1991, when standardized surveillance and reporting of Lyme disease began in the United States, case counts have increased steadily. Since 2007, more than 25,000 Lyme disease cases have been reported annually. Recent studies suggest that geographic location and seasonal climate variability may play a significant role in determining when and where Lyme disease cases are most likely to occur.
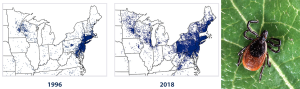
Figure 6 Details.
The reported cases of Lyme disease in 1996 and 2018 for the areas of the United States where Lyme disease is most common. (Source: CDC) In the eastern United States, Lyme disease is transmitted to humans primarily by blacklegged (deer) ticks. (Photo: S. Bauer)
A temperature relationship for sporadic local malaria transmission was observed in New York and New Jersey during the 1990s; common to these outbreaks was exceptionally hot and humid weather that reduced the development time of malaria sporozoites sufficiently to render these northern anopheline mosquitoes infectious.
The pulmonary hantavirus epidemic in the south-west of the USA was believed to be due to an upsurge in rodent populations related to climate and ecological conditions; six years of drought followed by extremely heavy spring rains in 1993 resulted in a 10-fold increase in the population of deer mice, which can carry hantavirus. Clusters of the disease have been spatially linked to areas with higher rainfall following El Niño events. Similarly, the incidence of flea-borne plague has been positively associated with preceding periods of heavy precipitation in the region.
Great Lakes Region and Climate Change
The Great Lakes contain 20% of the world’s surface freshwater, provide drinking water and livelihood to more than 35 million people, and allow for important economic and cultural services such as shipping and recreation. The Great Lakes influence regional weather and climate conditions and impact climate variability and change across the region. The lakes influence daily weather by 1) moderating maximum and minimum temperatures of the region in all seasons, 2) increasing cloud cover and precipitation over and just downwind of the lakes during winter, and 3) decreasing summertime convective clouds and rainfall over the lakes. In recent decades, the Great Lakes have exhibited notable changes that are impacting and will continue to impact people and the environment within the region. In particular, lake surface temperatures are increasing, lake ice cover is declining, the seasonal stratification of temperatures in the lakes is occurring earlier in the year, and summer evaporation rates are increasing.
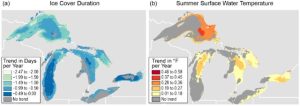
Figure 7 Details.
The duration of seasonal ice cover decreased in most areas of the Great Lakes between 1973 and 2013, while summer surface water temperature (SWT) increased in most areas between 1994 and 2013. (a) The map shows the rate of change in ice cover duration. (b) The map shows the rate of change in summer SWT. (Source: Mason et al. 2016)
Along the Great Lakes, lake-effect snowfall has increased overall since the early 20th century. However, studies have shown that the increase has not been steady, and it generally peaked in the 1970s and early 1980s before decreasing. As the warming in the Midwest continues, reductions in lake ice may increase the frequency of lake-effect snows until winters become so warm that snowfall events shift to rain.
Lake-surface temperatures increased during the period 1985–2009 in most lakes worldwide, including the Great Lakes. The most rapid increases in lake-surface temperature occur during the summer and can greatly exceed temperature trends of air at locations surrounding the lakes. From 1973 to 2010, ice cover on the Great Lakes declined an average of 71%; although ice cover was again high in the winters of 2014 and 2015, a continued decrease in ice cover is expected in the future.
Water levels in the Great Lakes fluctuate naturally, though levels more likely than not will decline with the changing climate. A period of low water levels persisted from 1998 to early 2013. A single warm winter in 1997–1998 (corresponding to a major El Niño event) and ongoing increases in sunlight reaching the lake surface (due to reduced cloud cover) were likely strong contributors to these low water levels. Following this period, water levels rose rapidly. Between January 2013 and December 2014, water levels on Lake Michigan-Huron rose by 1 m, at the highest rate ever recorded for a 2-year period beginning in January and ending in December of the following year.
This event coincided with below-average air temperatures and extensive winter ice cover across the Great Lakes. The rise in Lake Michigan-Huron levels in 2013 was due to above-average spring runoff and persistent over-lake precipitation. In 2014, it was due to a rare combination of below-average evaporation, above average runoff and precipitation, and very high inflow rates from Lake Superior through the St. Mary’s River. From January 18, 2013 (175.16 m a.s.l.) to September 8, 2020 (177.82 m a.s.l.), Lake Michigan-Huron experienced its fastest and highest rise (2.67 m) since 1860 when instrumental measurements began. In 2020 the Great Lakes had one of the warmest winters on record. The fourth lowest record for maximum ice cover on the lake was set in January and February. The Lake Michigan-Huron level was 94 cm above average at the end of February, and 38 cm higher than end of February 2019, setting record high levels for both January and February. Wet weather and very high lake levels (19 cm higher than in 2019) continued through the spring, when March, April, and May, monthly mean water level records were set on Lakes Michigan-Huron. Even though the summer was warmer than normal, higher than normal precipitation maintained lake levels 19 cm higher than 2019. Lake Michigan-Huron set new monthly mean record high water levels in June, July, and August. On September 8, 2020, Lake Michigan reached its highest level (177.823 m) in recorded history, and the second highest ever, after August 26, 1986 (177.837 m). However, warmer and drier weather in autumn caused Lake Michigan-Huron levels to drop 10 cm below their 2019 levels. During first four months of 2021 Lake Michigan-Huron levels were still higher than average, but monthly average levels were 19-35 cm lower than record high 2020 levels.
An important seasonal event for biological activity in the Great Lakes is the turnover of water, or destratification, which historically has occurred twice per year. Destratification occurs during the fall as the water temperature drops below a threshold of 4°C (39°F), the point at which freshwater attains its maximum density, and again during the spring when the water temperature rises above that threshold. The resultant mixing carries oxygen down from the lake surface and nutrients up from the lake bottom and into the water column. In a pattern that is similar to changes in duration of the growing season on land, the climate projections suggest that the overturn in spring that triggers the start of the aquatic “growing season” will happen earlier, and the fall overturn will happen later. This trend toward a longer stratified season has been documented at locations in Lake Superior. As the duration of the stratified period increases, the risk of impacts from low oxygen levels at depth and a lack of nutrient inputs at the surface increases, potentially leading to population declines of species in both zones.
Other examples include coastal wetland damage and disturbance by human structures that change habitat conditions and water flow patterns. Fish harvest and other management activities also have influences on populations. Especially in Lake Erie, runoff from agricultural watersheds can carry large volumes of nutrients and sediments that can reduce water quality, potentially leading to hypoxia (inadequate oxygen supply), an occurrence that is predicted to be more likely as the climate continues to change. Increased water temperatures and nutrient inputs also contribute to algal blooms, including harmful cyanobacterial algae that are toxic to people, pets, and many native species.
Climate change is expected to impact the species and fisheries of the Great Lakes. However, the vast size and low temperatures in these lakes suggest that mortality events from temperature are a much lower risk. One key aspect of the influence of warming lakes on fish growth is the availability of suitable thermal habitat, as ectotherms, or coldblooded species, can grow faster in warmer water due to temperature impacts on metabolic rates. Fish can behaviorally thermoregulate, meaning they can migrate to the portion of the water column that contains water of the particular species’ preferred temperature.
While some native fish may show enhanced growth, these same changes can influence the survival and growth of invasive species. Nonnative species such as alewife and zebra and quagga mussels have had dramatic impacts on the Great Lakes. Warmer conditions may lead to increases in invasion success and may increase the impact of invasive species that are already present. For example, sea lamprey are parasitic fish that are native to the Atlantic Ocean, and in the Great Lakes, they are the focus of several forms of control efforts. Climate change has potential to reduce the effectiveness of these efforts. In the Lake Superior watershed, in years with longer growing seasons (defined as the number of days with water temperatures above 50°F), lamprey reach larger weights before spawning. Larger body sizes suggest a greater impact on other fish species, because larger lamprey produce more eggs and require more food to survive.
Coastal communities and several economic sectors, including shipping, transportation, and tourism, are vulnerable to the changing climate impacts. While the most recent research underscores the great uncertainty in future lake levels, earlier research showed that scenarios of decreasing lake levels will increase shipping costs even if the shipping season is longer, or that lower ice cover could increase the damage to coastal infrastructure caused by winter storms.
This Chapter is compiled from following sources: Fourth National Climate Assessment, NASA’s Global Climate Change, NOAA’s Climate gov., NSIDC’s State of the Cryosphere, Marine Mammal Commission, World Wide Fund For Nature, Climate and Health Assessment, and World Health Organization.