36 Skeletal muscle metabolism
Learning Objectives
After reading this section you should be able to-
- Describe the sources of ATP (e.g., glycolysis, oxidative phosphorylation, creatine phosphate) that muscle fibers use for skeletal muscle contraction.
- Explain the factors that are believed to contribute to skeletal muscle fatigue.
- Compare and contrast the metabolism of skeletal muscle with that of cardiac and smooth muscle.
Glycolysis
Glucose is the body’s most readily available source of energy. After digestive processes break polysaccharides down into monosaccharides, including glucose, the monosaccharides are transported across the wall of the small intestine and into the circulatory system, which transports them to the liver. In the liver, hepatocytes either pass the glucose on through the circulatory system or store excess glucose as glycogen. Cells in the body take up the circulating glucose in response to insulin and, through a series of reactions called glycolysis, transfer some of the energy in glucose to ADP to form ATP (Figure 36.1). The last step in glycolysis produces the product pyruvate.
Glycolysis begins with the phosphorylation of glucose by hexokinase to form glucose-6-phosphate. This step uses one ATP, which is the donor of the phosphate group. Under the action of phosphofructokinase, glucose-6-phosphate is converted into fructose-6-phosphate. At this point, a second ATP donates its phosphate group, forming fructose-1,6-bisphosphate. This six-carbon sugar is split to form two phosphorylated three-carbon molecules, glyceraldehyde-3-phosphate and dihydroxyacetone phosphate, which are both converted into glyceraldehyde-3-phosphate. The glyceraldehyde-3-phosphate is further phosphorylated with groups donated by dihydrogen phosphate present in the cell to form the three-carbon molecule 1,3-bisphosphoglycerate. The energy of this reaction comes from the oxidation of (removal of electrons from) glyceraldehyde-3-phosphate. In a series of reactions leading to pyruvate, the two phosphate groups are then transferred to two ADPs to form two ATPs. Thus, glycolysis uses two ATPs but generates four ATPs, yielding a net gain of two ATPs and two molecules of pyruvate. In the presence of oxygen, pyruvate continues on to the Krebs cycle (also called the citric acid cycle or tricarboxylic acid cycle (TCA)), where additional energy is extracted and passed on.
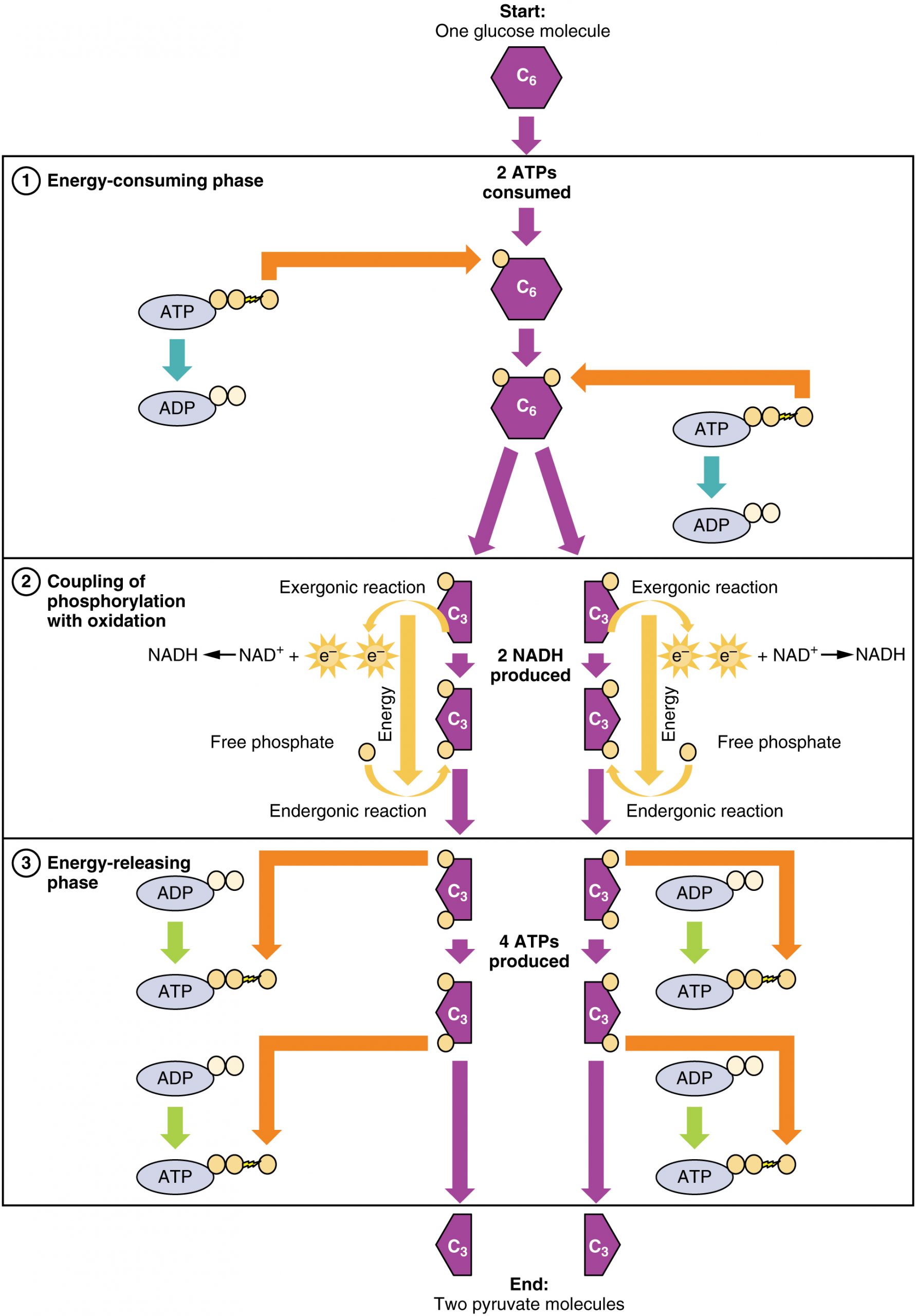
Glycolysis can be divided into two phases: energy consuming (also called chemical priming) and energy yielding. The first phase is the energy-consuming phase, so it requires two ATP molecules to start the reaction for each molecule of glucose. However, the end of the reaction produces four ATPs, resulting in a net gain of two ATP energy molecules.
Glycolysis can be expressed as the following equation:
Under anaerobic conditions, pyruvate is reduced to lactate, regenerating NAD⁺ for continued glycolysis
This equation states that glucose, in combination with ATP (the energy source), NAD+ (a coenzyme that serves as an electron acceptor), and inorganic phosphate, breaks down into two pyruvate molecules, generating four ATP molecules—for a net yield of two ATP—and two energy-containing NADH coenzymes. The NADH that is produced in this process will be used later to produce ATP in the mitochondria. Importantly, by the end of this process, one glucose molecule generates two pyruvate molecules, two high-energy ATP molecules, and two electron-carrying NADH molecules.
The second phase of glycolysis, the energy-yielding phase, creates the energy that is the product of glycolysis. Glyceraldehyde-3-phosphate dehydrogenase converts each three-carbon glyceraldehyde-3-phosphate produced during the energy-consuming phase into 1,3-bisphosphoglycerate. This reaction releases an electron that is then picked up by NAD+ to create an NADH molecule. NADH is a high-energy molecule, like ATP, but unlike ATP, it is not used as energy currency by the cell. Because there are two glyceraldehyde-3-phosphate molecules, two NADH molecules are synthesized during this step. Each 1,3-bisphosphoglycerate is subsequently dephosphorylated (i.e., a phosphate is removed) by phosphoglycerate kinase into 3-phosphoglycerate. Each phosphate released in this reaction can convert one molecule of ADP into one high-energy ATP molecule, resulting in a gain of two ATP molecules.
In summary, one glucose molecule breaks down into two pyruvate molecules, and creates two net ATP molecules and two NADH molecules by glycolysis. Therefore, glycolysis generates energy for the cell and creates pyruvate molecules that can be processed further through the aerobic Krebs cycle (also called the citric acid cycle or tricarboxylic acid cycle); converted into lactic acid or alcohol (in yeast) by fermentation; or used later for the synthesis of glucose through gluconeogenesis.
Aerobic Respiration
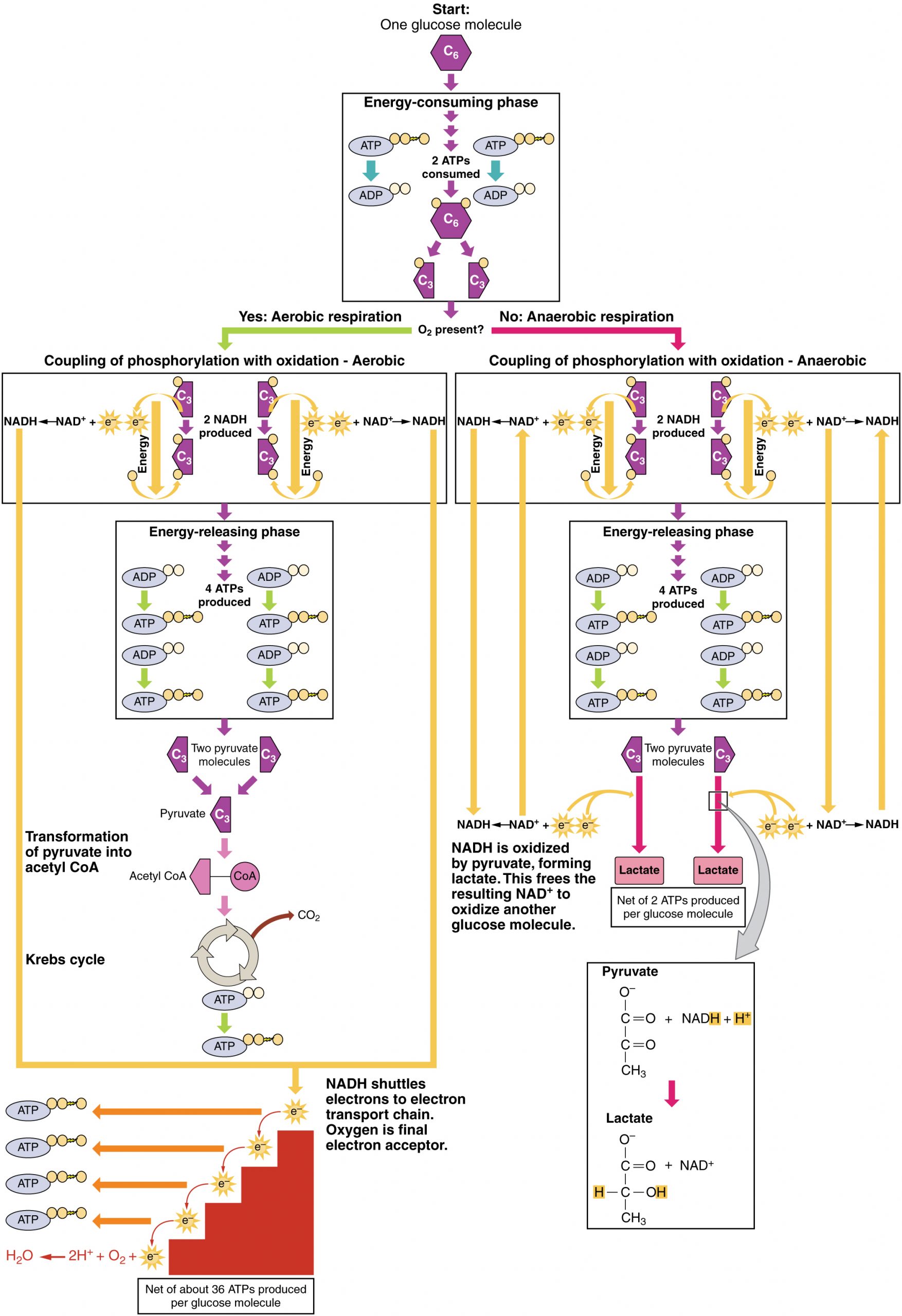
In the presence of oxygen, pyruvate can enter the Krebs cycle where additional energy is extracted as electrons are transferred from the pyruvate to the receptors NAD+, GDP, and FAD, with carbon dioxide being a “waste product” (Figure 36.2). The NADH and FADH2 pass electrons on to the electron transport chain, which uses the transferred energy to produce ATP. As the terminal step in the electron transport chain, oxygen is the terminal electron acceptor and creates water inside the mitochondria.
Krebs Cycle/Citric Acid Cycle/Tricarboxylic Acid Cycle
The pyruvate molecules generated during glycolysis are transported across the mitochondrial membrane into the inner mitochondrial matrix, where they are metabolized by enzymes in a pathway called the Krebs cycle (Figure 36.3). The Krebs cycle is also commonly called the citric acid cycle or the tricarboxylic acid (TCA) cycle. During the Krebs cycle, high-energy molecules, including ATP, NADH, and FADH2, are created. NADH and FADH2 then pass electrons through the electron transport chain in the mitochondria to generate more ATP molecules.
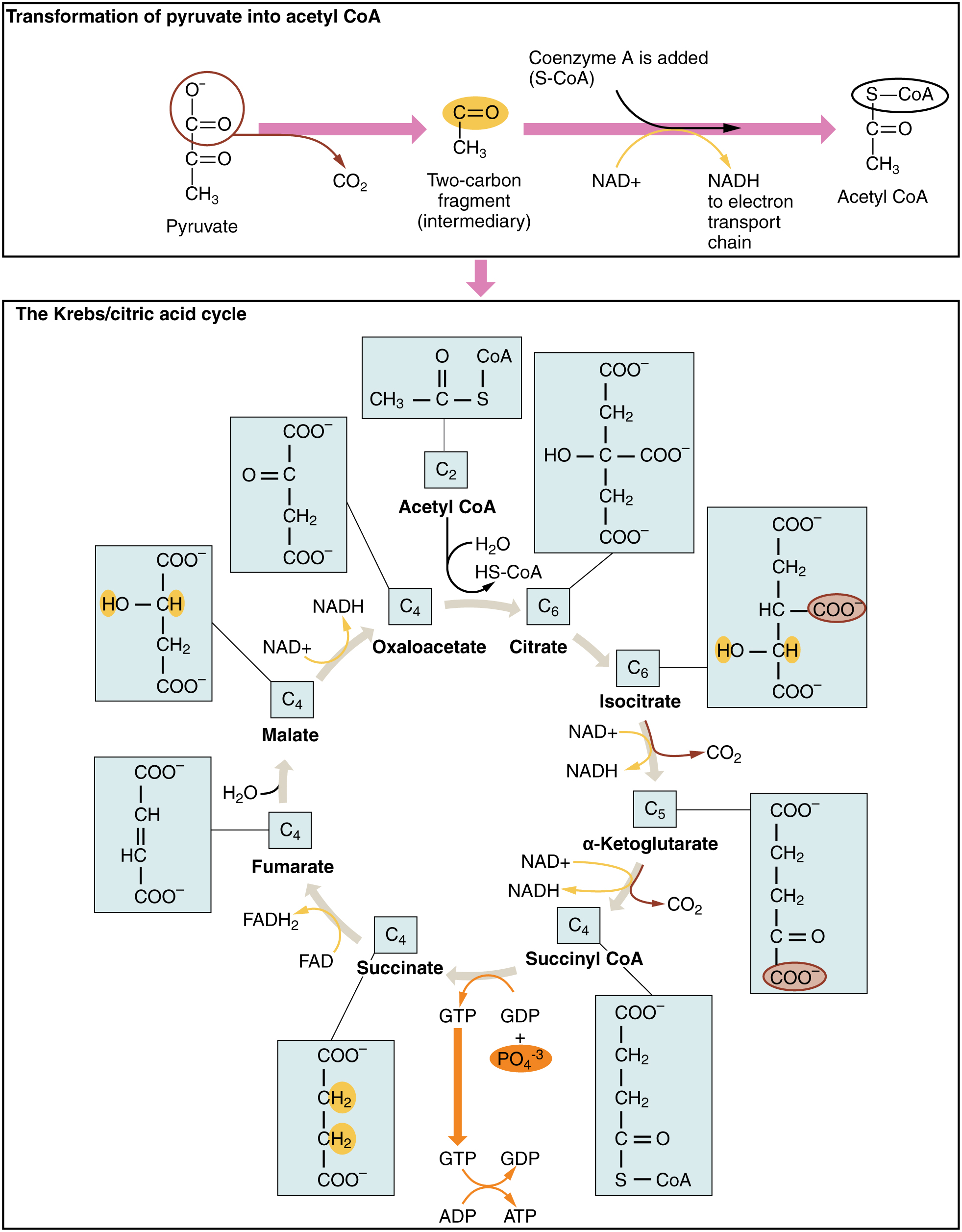
The three-carbon pyruvate molecule generated during glycolysis moves from the cytoplasm into the mitochondrial matrix, where it is converted by the enzyme pyruvate dehydrogenase into a two-carbon acetyl coenzyme A (acetyl CoA) molecule. This reaction is an oxidative decarboxylation reaction. It converts the three-carbon pyruvate into a two-carbon acetyl CoA molecule, releasing carbon dioxide and transferring two electrons that combine with NAD+ to form NADH. Acetyl CoA enters the Krebs cycle by combining with a four-carbon molecule, oxaloacetate, to form the six-carbon molecule citrate, or citric acid, at the same time releasing the coenzyme A molecule.
The six-carbon citrate molecule is systematically converted to a five-carbon molecule and then a four-carbon molecule, ending with oxaloacetate, the beginning of the cycle. Along the way, each citrate molecule will produce one ATP, one FADH2, and three NADH. The FADH2 and NADH will enter the oxidative phosphorylation system located in the inner mitochondrial membrane. In addition, the Krebs cycle supplies the starting materials to process and break down proteins and fats.
To start the Krebs cycle, citrate synthase combines acetyl CoA and oxaloacetate to form a six-carbon citrate molecule; CoA is subsequently released and can combine with another pyruvate molecule to begin the cycle again. The aconitase enzyme converts citrate into isocitrate. In two successive steps of oxidative decarboxylation, two molecules of CO2 and two NADH molecules are produced when isocitrate dehydrogenase converts isocitrate into the five-carbon α-ketoglutarate, which is then catalyzed and converted into the four-carbon succinyl CoA by α-ketoglutarate dehydrogenase. The enzyme succinyl CoA dehydrogenase then converts succinyl CoA into succinate and forms the high-energy molecule GTP, which transfers its energy to ADP to produce ATP. Succinate dehydrogenase then converts succinate into fumarate, forming a molecule of FADH2. Fumarase then converts fumarate into malate, which malate dehydrogenase then converts back into oxaloacetate while reducing NAD+ to NADH. Oxaloacetate is then ready to combine with the next acetyl CoA to start the Krebs cycle again (Figure 36.3). For each turn of the cycle, three NADH, one ATP (through GTP), and one FADH2 are created. Each carbon of pyruvate is converted into CO2, which is released as a byproduct of oxidative (aerobic) respiration.
Oxidative Phosphorylation and the Electron Transport Chain
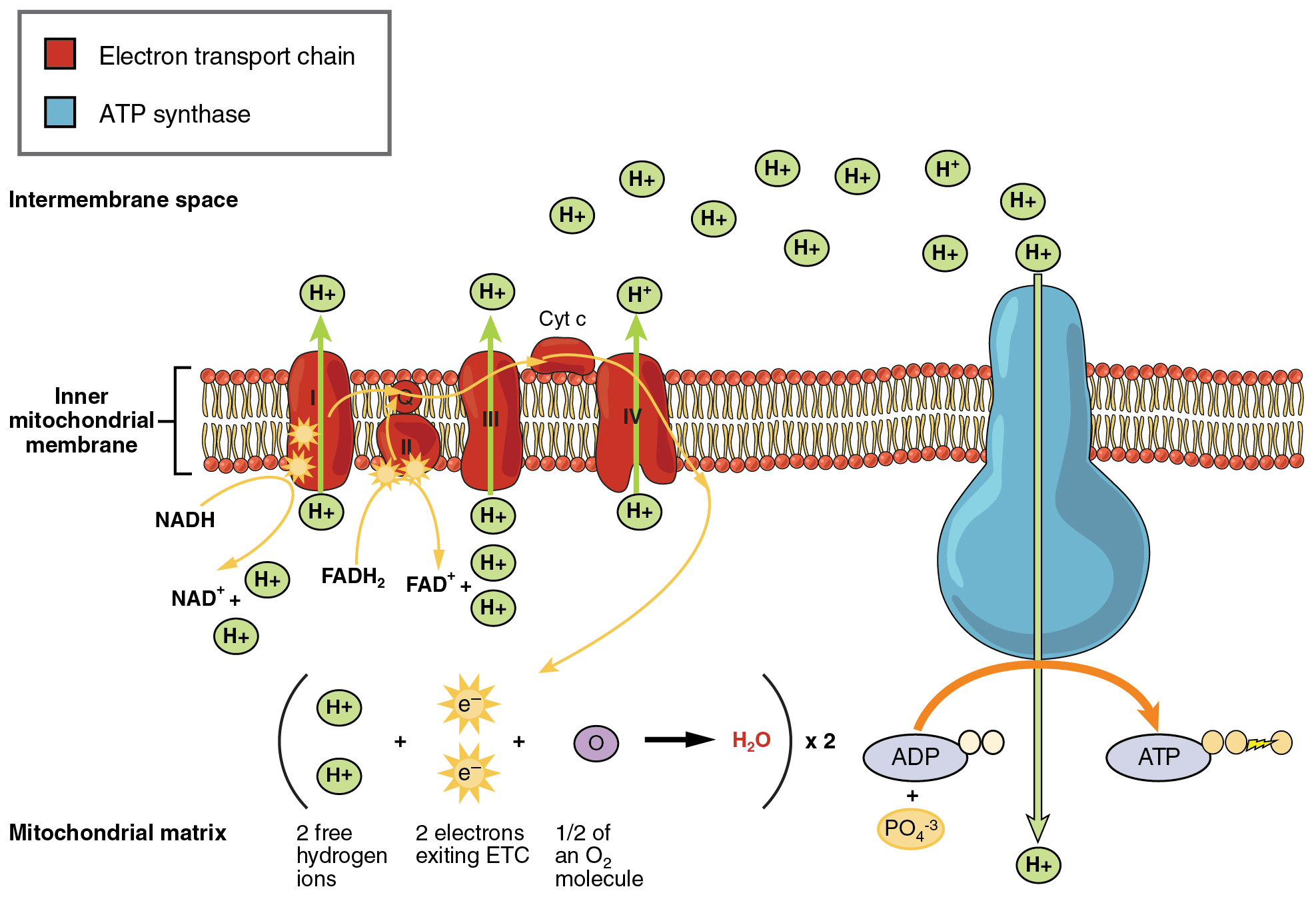
The electron transport chain (ETC) uses the NADH and FADH2 produced by the Krebs cycle to generate ATP. Electrons from NADH and FADH2 are transferred through protein complexes embedded in the inner mitochondrial membrane by a series of enzymatic reactions. The electron transport chain consists of a series of four enzyme complexes (Complex I – Complex IV) and two coenzymes (ubiquinone and Cytochrome c), which act as electron carriers and proton pumps used to transfer H+ ions into the space between the inner and outer mitochondrial membranes (Figure 36.4). The ETC couples the transfer of electrons between a donor (like NADH) and an electron acceptor (like O2) with the transfer of protons (H+ ions) across the inner mitochondrial membrane, enabling the process of oxidative phosphorylation. In the presence of oxygen, energy is passed, stepwise, through the electron carriers to collect gradually the energy needed to attach a phosphate to ADP and produce ATP. The role of molecular oxygen, O2, is as the terminal electron acceptor for the ETC. This means that once the electrons have passed through the entire ETC, they must be passed to another, separate molecule. These electrons, O2, and H+ ions from the matrix combine to form new water molecules. This is the basis for your need to breathe in oxygen. Without oxygen, electron flow through the ETC ceases.
Creatine phosphate
ATP supplies the energy for muscle contraction to take place. In addition to its direct role in the cross-bridge cycle, ATP also provides the energy for the active-transport Ca2+ pumps in the SR. Muscle contraction does not occur without sufficient amounts of ATP. ATP is a relatively unstable molecule and storing large amounts for any amount of time is not possible. Because the amount of ATP stored in muscle is very low, only sufficient to power a few seconds worth of contractions. As it is broken down, ATP must therefore be regenerated and replaced quickly to allow for sustained contraction. There are three mechanisms by which ATP can be regenerated: creatine phosphate metabolism, anaerobic glycolysis, fermentation and aerobic respiration.
Creatine phosphate is a molecule that can store energy in its phosphate bonds and is more stable than ATP. In a resting muscle, excess ATP transfers its energy to creatine, producing ADP and creatine phosphate. This acts as an energy reserve that can be used to quickly create more ATP. When the muscle starts to contract and needs energy, creatine phosphate transfers its phosphate back to ADP to form ATP and creatine. This reaction is catalyzed by the enzyme creatine kinase and occurs very quickly; thus, creatine phosphate-derived ATP powers the first few seconds of muscle contraction. However, creatine phosphate can only provide approximately 15 seconds worth of energy, at which point another energy source has to be used (Figure 36.5).
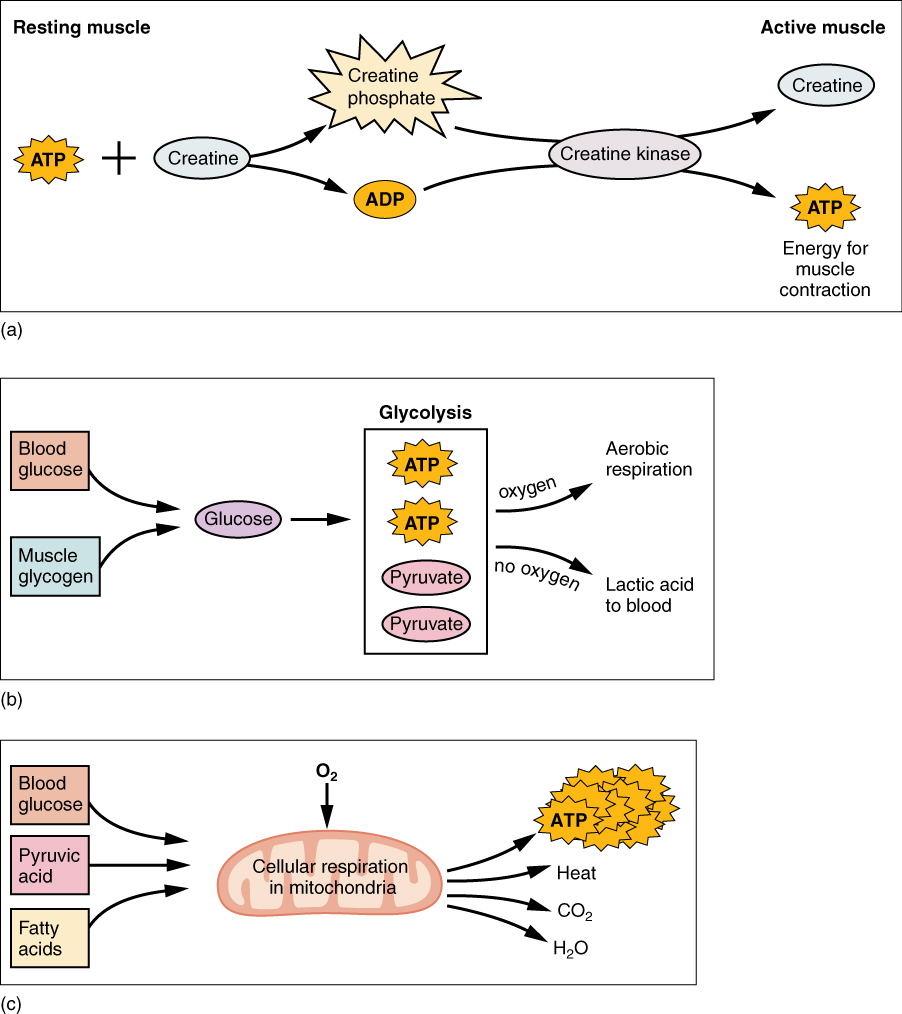
Muscle fatigue
Muscle fatigue refers to the decline in the muscle’s ability to generate force or power, which can result from prolonged or intense activity. It is a complex phenomenon influenced by various factors, which can be broadly categorized into central and peripheral fatigue.
Peripheral Fatigue
Peripheral fatigue originates within the muscle itself and involves several mechanisms that impair the muscle’s contractile function and energy production:
- Metabolic Byproducts: Accumulation of metabolic byproducts such as lactic acid, inorganic phosphate, and hydrogen ions during intense exercise can lower the pH within the muscle. This acidic environment can inhibit key enzymes involved in ATP production and interfere with the contractile proteins, actin and myosin, reducing their ability to generate force.
- Depletion of Energy Stores: Prolonged muscle activity depletes ATP and creatine phosphate reserves, reducing the immediate energy available for contraction. Additionally, glycogen stores can become depleted, limiting the substrate availability for glycolysis and oxidative phosphorylation.
- Impaired Calcium Handling: Efficient muscle contraction relies on the release and reuptake of calcium ions by the sarcoplasmic reticulum. Fatigue can disrupt this calcium cycling, resulting in less calcium being available to bind to troponin and initiate contraction, thereby reducing muscle force production.
- Damage to Muscle Fibers: Repeated or high-intensity contractions can cause structural damage to muscle fibers, including disruptions to the sarcolemma (muscle cell membrane) and damage to the sarcoplasmic reticulum. This damage can impair the muscle’s ability to contract and recover.
Central Fatigue
Central fatigue involves the central nervous system (CNS) and refers to a reduction in the neural drive or motor output to the muscles:
- Neural Inhibition: The CNS may reduce the neural drive to the muscles as a protective mechanism to prevent damage. This can be influenced by sensory feedback from the fatigued muscles, indicating discomfort or potential injury.
- Neurotransmitter Depletion: Prolonged exercise can lead to the depletion of neurotransmitters such as acetylcholine at the neuromuscular junction, impairing the transmission of action potentials from motor neurons to muscle fibers—reducing the size of each end-plate potential and making muscle action potentials harder to trigger.
- Psychological Factors: Mental fatigue, motivation, and perceived exertion play significant roles in central fatigue. Factors such as stress, anxiety, and lack of motivation can decrease the willingness or ability to sustain muscle activity.
Interactions Between Central and Peripheral Fatigue
Central and peripheral fatigue often interact and influence each other. For example, the accumulation of metabolic byproducts in the muscle can send feedback to the CNS, contributing to central fatigue by signaling discomfort or the need to reduce activity. Conversely, central fatigue can lead to decreased motor output, which reduces muscle activation and contributes to the development of peripheral fatigue.
Comparing Metabolism in Skeletal, Cardiac, and Smooth Muscle
- Skeletal Muscle: Relies on glycolysis, oxidative phosphorylation, and creatine phosphate for ATP. It has a high capacity for anaerobic metabolism, making it well-suited for rapid, intense contractions.
- Cardiac Muscle: Primarily uses oxidative phosphorylation for ATP production, relying heavily on a continuous oxygen supply. It has abundant mitochondria and a steady supply of oxygen via coronary circulation, enabling it to sustain prolonged contractions without fatigue.
- Smooth Muscle: Utilizes a mix of oxidative phosphorylation and glycolysis. It can function effectively in low-oxygen environments and is adapted for slow, sustained contractions with low fatigue rates.
Adapted from Anatomy & Physiology by Lindsay M. Biga et al, shared under a Creative Commons Attribution-ShareAlike 4.0 International License, chapters 10, 24.
the anaerobic metabolic pathway occurring within the cytoplasm of a cell that splits a molecule of glucose into two pyruvate molecules
the intermediate of metabolic pathways, formed from glycolysis
also known as the citric acid cycle and/or tricarboxylic acid cycle; the sequence of chemical reactions in the mitochondrial matrix that oxidize acetyl coenzyme A and produce NADH, FADH2, and ATP
the stage of glycolysis in which ATP is used to break glucose down into two three-carbon molecules
the second phase of glycolysis, during which ATP is produced
metabolic process occurring in the liver and kidneys during which non-carbohydrate sources are utilized to form glucose
oxygen in the electron transport chain; the last compound to receive an electron from another compound
a molecule that participates in the Krebs Cycle by providing acetyl groups to be oxidized
a series of chemical reactions that occur in the inner mitochondrial membrane in which electrons are moved across the membrane and ATP is produced
the process of ATP formation through the transfer of electrons by electron carriers
muscle fatigue originating in the muscle due to depletion of energy stores, a buildup of metabolic byproducts, impaired calcium release, damage to muscle fibers, and/or central fatigue mechanisms
muscle fatigue originating in the central nervous system due to psychological factors, neurotransmitter depletion, neural inhibition, and/or peripheral factors